Assisted sexual reproduction of Acropora cervicornis for active restoration on Florida’s Coral Reef
- 1Coral Reef Restoration Program, The Elizabeth Moore International Center for Coral Reef Research and Restoration, Mote Marine Laboratory, Summerland Key, FL, United States
- 2Coral Reef Monitoring and Assessment Program, The Elizabeth Moore International Center for Coral Reef Research and Restoration, Mote Marine Laboratory, Summerland Key, FL, United States
Given the rapid, global decline in the health and abundance of coral reefs, increased investments in restoration-based interventions -including asexual and sexual propagation- are being made by coral reef scientists at research institutions, but also at zoos and aquariums. Mote Marine Laboratory & Aquarium is an independent, non-profit marine science organization dedicated to the conservation and restoration of Florida’s Coral Reef, and does so, using science-based strategies. In order to promote the long-term persistence, resilience, and adaptive potential of restored coral populations on Florida’s Coral Reef, Mote scientists are performing critical research and restoration activities related to assisted sexual reproduction (ASR). The objective of this study was to optimize ASR of Acropora cervicornis by (1) evaluating broodstock compatibility for genets actively used within Mote’s restoration gene pool, (2) optimizing larval settlement by testing spectral cues, (3) and optimizing the grow-out of sexual recruits by testing the impact of light on growth, survival, and algal symbiont uptake in the presence of adult corals or not. Overall, we found that corals and genets spawned with high synchrony, both within and across years, and in terms of predicted spawning times related to nights after the full moon and minutes after sunset. Across two years, overall fertilization success was high (~95%), but we did find one pair of genets that was not compatible. During settlement, larvae preferred pink and purple-colored substrates, which was consistent with our expectation that they would select substrates similar in color to crustose coralline algae (CCA). Interestingly though, they only did so when a matching chemical cue from CCA was also present, indicating that larvae integrate multiple cues simultaneously to determine the most appropriate place to settle. Growth and symbiont uptake were faster in recruits reared in the presence of adult corals and additional lighting, but survivorship was not different through the first ten weeks post-settlement between treatments. A subset of corals was outplanted using two different techniques based on single or clustered corals. We report the initial 1-month survival results. We also provide a detailed protocol and general recommendations for ASR based on years of coral sexual propagation experience.
1 Introduction
Over the last four decades, Florida’s Coral Reef has suffered dramatic declines in the health and abundance of its most valuable stony coral species as a result of local and global stressors (Williams et al., 2008; Eakin et al., 2010; Colella et al., 2012; van Woesik et al., 2014; Kuffner et al., 2015; Precht et al., 2016; NCCOS, 2018; Walton et al., 2018). Almost 90% of the live corals that once dominated this reef system have been lost and coral cover is now estimated to be as low as 2-6% (Donovan et al., 2020). As such, increased investments are being made to conserve what is left while actively restoring what has been lost (Boström-Einarsson et al., 2020). For example, the National Oceanic and Atmospheric Association (NOAA) is leading a large-scale, multi-million-dollar collaborative effort to restore scleractinian corals at seven ecologically and culturally significant reef sites in the Florida Keys, an initiative called Mission: Iconic Reefs (NOAA, 2020).
Contributing to this effort, and to the restoration of the entire reef tract, are zoos and aquariums, which have become key players in the holding, propagation, and outplanting of threatened coral species, as well as locations where science-based restoration strategies are being developed and applied. For example, at Mote Marine Laboratory and Aquarium’s facility in the Florida Keys, The Elizabeth Moore International Center for Coral Reef Research and Restoration (IC2R3), scientists are conducting research on coral health and disease, stress tolerance, ocean acidification, restoration science, and reproduction science. At IC2R3, the Coral Restoration Program carries out a comprehensive framework for active restoration of Florida’s Coral Reef, which includes interventions related to resilience/resistance screening, asexual propagation, assisted sexual reproduction (ASR), outplanting, population and ecosystem monitoring, gene banking, and field- and land-based coral nursery management. This multi-faceted approach works to promote rapid population and reef recovery by integrating asexual and sexual propagation techniques to quickly upscale the number and diversity of stress-tolerant corals that can be used for restoration. The ultimate goals are to rapidly increase coral cover and get restored populations to a sexually mature self-sustaining state as quickly as possible, and with enough genotypic and phenotypic variation, so that they can effectively respond to changing environmental conditions (Baums et al., 2019).
Assisted sexual reproduction (ASR) of corals is a rapidly growing field within coral restoration science (Marhaver et al., 2017b; Calle-Trivino et al., 2018; Randall et al., 2020), and especially in Florida where natural sexual cycles appear to be failing for some species (Baums et al., 2019). Sexual reproduction is necessary for the long-term persistence of species and can increase the adaptive potential and resilience of populations by promoting genetic diversity (Baums et al., 2019). Generally, sexual reproduction in stony corals involves the following processes: gametogenesis, spawning, fertilization, embryogenesis, planulation (formation of planulae larvae), settlement, and recruitment where metamorphosed individuals become established within the reef community (Harrison, 2011). However, persistent recruitment failure has been reported for several species in Florida (Hughes and Tanner, 2000; Williams et al., 2008; van Woesik et al., 2014; Miller et al., 2018; Baums et al., 2019). It is hypothesized that wild populations are too small or not dense enough to support successful fertilization (e.g., sperm limitation and Allee effects) (Levitan et al., 2004; Levitan et al., 2011). This could be one explanation for why we are failing to see new generations of sexual recruits show up on Florida reefs. If populations are no longer self-sustaining through natural sexual cycles, the benefits of sexual reproduction may be lost (e.g., replenishment of depleted adult populations, population recovery post-disturbance, gene flow, and increased genetic variation).
As such, scientists and practitioners are stepping in to carry out coral sexual cycles in the laboratory to ensure the benefits of sexual reproduction are realized for restored populations. However, ASR and its associated processes can be somewhat species-specific. Thus, these processes require optimization in order to upscale and promote coral growth and survival, while at the same time reducing the time from production to restoration, which can take anywhere from months to years depending on the species and how soon sexually produced corals are put back out onto reefs. Therefore, the purpose of this study was to carry out ASR of A. cervicornis (Figure 1), while optimizing upscaled processes related to spawning, fertilization, settlement and grow-out with the overall intention to effectively generate a large number of diverse corals to be used for active restoration.
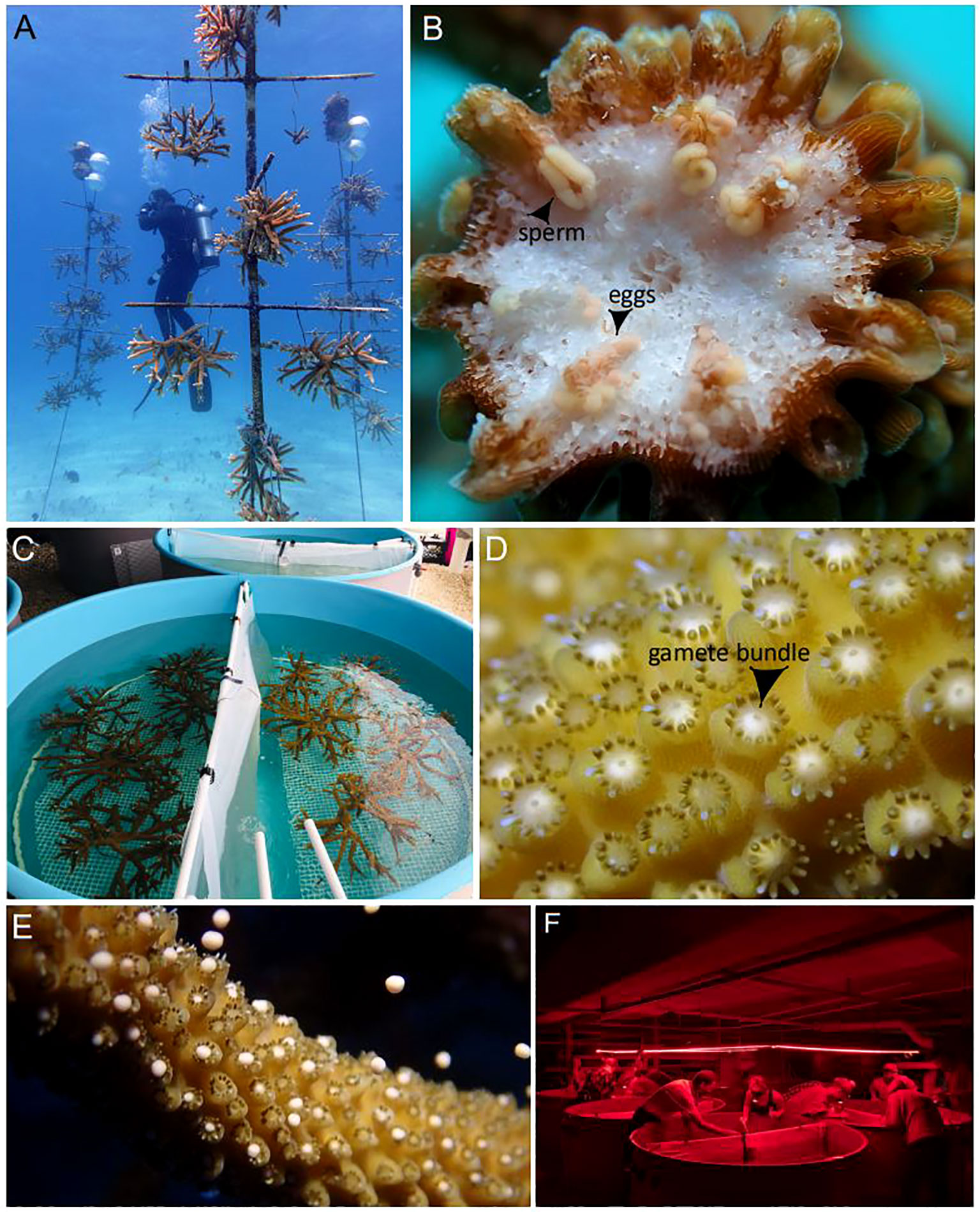
Figure 1 Assisted sexual reproduction of A. cervicornis. (A) Offshore coral spawning nursery; each coral tree holds replicate colonies of a single genet. (B) Sexual maturity assessment; in the weeks preceding the predicted annual reproduction event, corals were sampled to confirm they were gravid (i.e., contain eggs (pink-orange spheres) and sperm (cream-colored packets)). (C) Ex-situ spawning setup; a few days before the full moon, corals were transferred from the in-situ nursery to the laboratory where they were held in large outdoor mesocosms that had access to sunset and lunar cues. (D) Coral setting; gamete bundles appeared in the polyps’ mouths indicating spawning was imminent. (E) Coral spawning; buoyant gamete bundles float to the surface. (F) Gamete bundle collection; bundles were collected using red lights to prevent light pollution from disrupting spawning. Source: (A) Sarah Hamlyn; (B-E) Hanna Koch; (F) Haley Burleson.
For this study, we used A. cervicornis because it was once a dominant species in Florida, but has since experienced a greater than 95% population reduction (Acropora Biological Review Team, 2005). Major losses are attributed to bleaching, disease, storms, cold snaps, and direct anthropogenic impacts (Gardner et al., 2005; Hemond and Vollmer, 2010; Gignoux-Wolfsohn et al., 2012; Enochs et al., 2014; Drury et al., 2016; Kemp et al., 2016; NCCOS, 2018; Gignoux-Wolfsohn et al., 2019; Goergen et al., 2019). Historically, this species has been the target of large-scale asexual restoration efforts owing to the critical ecosystem services it provides in terms of habitat and shelter to other reef organisms (Levy et al., 2010; Lirman et al., 2010; NMFS, 2015; Lohr and Patterson, 2017; Schopmeyer et al., 2017). Only more recently has it become a target for ASR. Furthermore, several genetic varieties (‘genets’) within Mote’s restoration gene pool have already been screened for increased resilience to heat stress and/or resistance to disease (Muller et al., 2018). This information is being fed into breeding designs based on controlled, two-parent crosses for evaluating the potential to conduct selective breeding based on certain desirable phenotypes (van Oppen et al., 2015; Koch, 2021b). These evaluations are beyond the scope of the present study, but the production of these families (i.e., crosses) was conducted herein as part of the ASR optimization process.
Specific ASR research aims herein were based on (1) evaluating broodstock compatibility in terms of spawning synchrony and fertilization success across multiple genets and years, (2) testing spectral cues associated with substrate color for optimizing settlement, and (3) optimizing the grow-out of sexual recruits by evaluating the impact of additional light and the presence of adult corals during early post-settlement months. To bolster our results and demonstrate reproducibility for certain aspects of the ASR process, we targeted two annual reproduction events corresponding to 2020 and 2021. Our hypotheses are as follows.
In terms of broodstock compatibility and spawning synchrony, we predicted that A. cervicornis would spawn within 1-15 nights after the August full moon, and have a peak spawning window where the most corals/genets would release gametes around nights 3-6 and 125-200 minutes after sunset (Jordan, 2018). We also expected to observe the same colonies spawn over multiple consecutive nights (Jordan, 2018). In terms of broodstock compatibility and fertilization, we hypothesized that gametic or genotypic incompatibilities may occur since such observations, including for acroporids, have previously been reported (Fogarty et al., 2012; Baums et al., 2013; Miller et al., 2018).
Regarding settlement, it is well documented that chemical cues given off by certain species of crustose coralline algae (CCA) act as an inducing mechanism for coral larval settlement and metamorphosis (Erwin et al., 2008; Tebben et al., 2015; Elmer et al., 2018; Whitman et al., 2020; Jorissen et al., 2021). Less studied is the role spectral cues (e.g., color) may play in coral settlement (Mason et al., 2011; Foster and Gilmour, 2016). We hypothesized that coral larvae would prefer to settle on substrates similar in color to CCA (i.e., pink and purple). If this were the case, we may be able to increase settlement rates, and thus, upscale the number of offspring produced simply by replacing standard white substrates with CCA-colored ones.
Finally, we predicted that sexual recruits would uptake algal symbionts (‘zooxanthellae’) faster, grow faster, and have greater survivorship when reared in the presence of adult corals and additional lighting. Typically, broadcast spawning species have aposymbiotic larvae; zooxanthellae are acquired early post-settlement via horizontal transmission from the surrounding seawater (Coffroth et al., 2006; Baird et al., 2009). While this process happens naturally in a land-based nursery with a flow-through system utilizing natural seawater, it can be slow and take weeks to months to achieve full uptake. Because algal symbionts (Family: Symbiodiniaceae) provide more than 90% of the coral’s daily metabolic energy requirements via photosynthetic activity (Muscatine et al., 1981), establishing this symbiosis early and rapidly is important for survival and growth. One strategy predicted to promote faster symbiont uptake is to rear settlers with adult corals (Nitschke et al., 2016; Quigley et al., 2018; Ali et al., 2019; Randall et al., 2020) that naturally shed algal symbionts in their epidermal mucocyte cells (Brown and Bythell, 2005). Once symbiosis is established, host energetic demands increase, and corals require more light to grow and survive. However, sensitive early life stages are oftentimes reared in indoor laboratories where conditions are more stable and controlled, but ambient light levels may be naturally low and necessitate the use of artificial lights to meet the coral’s increased energetic needs. If additional lighting supports faster growth, then recruit grow-out time could be reduced, along with effort, resources, time and monies.
How long corals are maintained in a nursery before outplanting, as well as how corals are outplanted, depends on many factors including project goals and availability of time, skilled personnel, space, and resources. Putting corals out sooner is faster and cheaper, but survival may be lower because mortality rates of sexual recruits reduce with size (Vermeij and Sandin, 2008; Speare et al., 2022). For this reason, Mote’s strategy is to grow corals (sexual recruits or microfragments) to a larger size (~2 ½ cm) before outplanting them to promote survival and retention of diversity. Herein, we used the 2020 cohort for active restoration in the Lower Florida Keys and did so utilizing two different outplanting strategies (i.e., outplanted genets either as single plugs or clusters of clonal fragments). While reporting on the long-term differences between these two strategies is beyond the scope of this study, we discuss preliminary 1-month post-outplant survival results and potential costs/benefits associated with the different strategies.
2 Methods and materials
2.1 Coral spawning nurseries
Two offshore A. cervicornis spawning nurseries (Figure 1A), ~48 km apart in the Lower Florida Keys, were created in 2019 and within Mote’s existing coral nurseries at Looe Key and Sand Key. Our nursery consisted of coral trees, which are mid-water floating structures tethered to the seafloor by a duckbill anchor, buoyed via sub-surface floats, and composed of a 183 cm vertical PVC trunk with five 91 cm horizontal fiberglass arms. Trees held up to ten replicate colonies of large size (up to ~60 cm diameter) that were hung from each arm by two attachment points using 136 kg capacity monofilament and aluminum sleeves (Figure 1A). Sexual maturity in scleractinian corals is size-dependent (Szmant, 1986; Babcock, 1991; Soong and Lang, 1992), with the predicted puberty size of this species to be ~25-30 cm diameter (Schopmeyer et al., 2017; Foster and Gilmour, 2020), or ~5,000-10,000 cm3 if ellipsoid volume is used as a size metric (Kiel et al., 2012; Huntington and Miller, 2014). The formula to convert from linear dimensions to colony volume is as follows:
Because larger corals tend to be more fecund (Hall and Hughes, 1996; Sakai, 1998; Beiring and Lasker, 2000; Elahi and Edmunds, 2007; Nozawa and Lin, 2014; Foster and Gilmour, 2020), we let the colonies grow to 2-3x the size needed for sexual maturity in order to maximize access to as many propagules as possible for upscaling ASR. Colonies began as asexual fragments, ~15 cm in diameter, that were harvested from the existing coral nursery at each location, which are used for asexual restoration. The spawning nursery was maintained as needed throughout the year by manually cleaning the trees and removing fouling organisms. The genets held within each spawning nursery were as follows: Looe Key (1, 3, 7, 13, 31, 34, 41, 44, 50, 62); Sand Key (1, 3, 7, 13, 31, 34, 41, 44, 47, 50, 62). All genets were originally sourced from the wild (i.e., natural populations in the Lower Florida Keys).
2.2 Morphometric and sexual maturity assessments
A. cervicornis has an annual reproductive event that typically follows the August full moon in Florida (Jordan, 2018). Gametogenesis in acroporids begins shortly after each annual reproduction event, with oogenesis typically beginning in November and spermatogenesis in January (Vargas-Angel et al., 2006). In the months preceding the spawning event, oocytes become visible by eye, but are relatively small and unpigmented (i.e., Stages II/III). However, within the last lunar cycle leading up to gamete release, oocyte size and pigmentation dramatically increase (i.e., Stages III/IV) (Vargas-Angel et al., 2006), and male gametes become visible as the sperm cells are assembled into discrete packets (i.e., Stage IV). It is then straightforward to identify gravid (pregnant) corals in-situ simply by breaking off a branch of the colony and looking inside the fragment to see if eggs and sperm are visible (Figure 1B). Thus, the size and pigmentation of gametes are strong indicators of whether the coral is expected to spawn in the upcoming lunar cycle.
Herein, we assessed the reproductive status of all genets present in each of Mote’s spawning nurseries before each predicted spawning event by sampling up to three replicate branches per replicate colony per genet (Figure 1B). Not every branch in a colony may develop gametes so replicate sampling may be necessary to confirm a gravid state. However, once a gravid branch was obtained, we stopped sampling to prevent unnecessary oversampling. The most recent growth typically does not develop gametes [i.e., sterile zones; (Wallace, 1985; Soong and Lang, 1992)], so we sampled branches ~10-12 cm in length and from the central portion of the colony (Foster and Gilmour, 2020). Colony morphometric data were collected for every sampled coral (Table S1). For obtaining samples, we targeted corals that were apparently healthy and above the predicted puberty size. Fragments were visually inspected for the presence of gametes and results recorded (Table S1).
2.3 Ex-situ spawning and gamete collections
Corals were brought to IC2R3 a few days before the August (2020) and July (2021) full moon for ASR. In 2020, we transported corals (52 colonies, 10 genets) from the Sand Key spawning nursery to the laboratory in large bins filled with seawater. In 2021, we transported corals (17 colonies, 9 genets) from the Looe Key spawning nursery to the laboratory without being in seawater (Figure S1). If done properly (see Figure S1 for description), corals can be transported for short durations (e.g., 30-45 minutes) without being submerged in seawater, and without any negative impacts to coral health or reproduction.
Back at the laboratory, corals were placed into fiberglass, flow-through, 1,800 L circular (183 cm diameter) mesocosms (Figure 1C) filled with ambient (~28°C) seawater pumped in from the adjacent canal. All seawater on site was treated with mechanical filtration and UV sterilization. Air wands maintained the pH of the seawater around 8 and pumps (Maxspect Xf350 Gyre Pump) were used to maintain circulation. Flow rates were ~6 L/min. Each mesocosm held replicate colonies of two genets. During spawning, we used mesh barriers to prevent gamete bundle mixing between the two genets held within a single mesocosm (Figure S2). Mesocosms were outside so corals had access to sunset and lunar cues. To prevent light pollution from disrupting spawning, all external building lights were shut off at sunset each night. Water and air in the mesocosms were turned off ~1hr before the predicted spawning time.
In 2020 and 2021, we monitored the corals for the first seven and nine nights after the full moon, respectively. Starting at 9pm each night, (~1hr after sunset) we monitored the corals at 30-min intervals to check for gamete bundle setting where the bundles are moved into the polyps’ mouths, become visible by eye, and indicate spawning is imminent (Figure 1D). Once this happened, we monitored the corals continuously until spawning commenced (Figure 1E). We used red headlamps (Figure 1F) until all colonies that had set started spawning, at which time we switched to white lights. For every spawned colony, we recorded the time setting started, the time spawning started/ended, as well as the percentage of total colonies that spawned (Table 1). We collected gamete bundles at the water’s surface using transfer pipettes and placed them into 50 mL centrifuge tubes. We collected gamete bundles for ~30 minutes and stopped before bundle dissolution.
2.4 In-vitro fertilization and controlled two-parent crosses
Gamete bundles were taken into the laboratory, which was previously cleaned, sterilized (using 70% EtOH) and ventilated. Equipment/supplies were also previously acid washed (10% muriatic acid) or bleached (10% bleach). All plastics used were made of polystyrene. Gamete bundles sat at room temperature (28°C) for 30-60 min to allow dissolution (Figure S3A), after which time we poured each collection over a sieve (~50 µm) to separate sperm and eggs. Sperm flowed through the sieve into a fertilization container (Norpro 4-Cup Gravy Fat Separator), while the eggs remained on the sieve (Figure S3B). We washed the eggs by submerging the sieve in ultra-filtered seawater (U-FSW) (0.7µm Whatman 1825-047 Glass Filter) in a 600 mL glass beaker. We did this four times, or until the water was no longer cloudy. Using a 500 mL squeeze bottle filled with U-FSW, we washed the eggs into a collection cup (4 oz. sterile specimen cup) that also had a very thin layer of U-FSW at the bottom. We did this to create separate sperm and egg stocks for every genet (Figure S3C).
In 2020 and 2021, we conducted 29 and 20 unique two-parent controlled crosses, respectively. Some failed crosses in 2020 were repeated in 2021 to test for reproducibility (see Results). We carried out replicate fertilization assays under standardized, controlled conditions including ambient temperature (28°C), U-FSW, sperm concentration of ~106 cells/mL (Oliver and Babcock, 1992; Nozawa et al., 2015), no more than a single layer of eggs on the surface of each container, and for a duration of ~60min (Marhaver et al., 2017b) (Figure S3D). We used a hemocytometer and compound microscope to obtain replicate cell counts of sperm stocks. The target concentration was achieved by diluting the sperm stocks with U-FSW. Fertilization was initiated by gently pouring the eggs down the inside of the fertilization containers with the diluted sperm. Assays were stirred using a transfer pipet at 0 and 30min. After 60min, the seawater in the fertilization container was slowly poured out, making sure to keep the buoyant eggs on the surface. This was followed by re-filling the container with U-FSW through the spout and letting it sit for ~30sec to allow the eggs to float to the surface. We repeated the rinsing process at least four times, or until the water was no longer cloudy (Figure S3E). Afterwards, we transferred the eggs to plastic deli containers (20 x 20 x 8 cm) with ~1 L of U-FSW. Each container held a few thousand eggs (Figure S4A) and was left on the bench at room temperature overnight.
The next morning, we scored fertilization success of each culture by using a dissecting microscope to enumerate the number of fertilized and unfertilized eggs in replicate sub-samples. Fertilized eggs were distinguished from unfertilized eggs by their morphology, whereby 11-13 hpf (hours post-fertilization), developing embryos had reached the ‘prawn chip’ stage, which has a very distinct irregular shape (Figures S4C, D). Unfertilized eggs remained spherical (Figure S4C, D). Within a day, the unfertilized eggs had degraded and were manually removed using transfer pipettes (Figure S4B). We maintained clean cultures by transferring embryos to new bins with fresh seawater every other day (Marhaver et al., 2017a).
In 2021, we conducted two types of selfing controls. For every genet, we conducted replicate (n=3) ‘No Sperm’ and ‘Sperm + Egg’ assays. For the No Sperm assays, we placed 50 gamete bundles in a 50 mL centrifuge tube, allowed bundle dissolution, and then separated eggs and sperm as previously described. The remaining rinsed eggs were transferred to large petri dishes with U-FSW and sat at room temperature overnight. For the Sperm + Egg assays, we processed 100 gamete bundles in the same way and after separation, the gametes were combined together in a large petri dish with the same target sperm concentration. After 60min, we rinsed the eggs and transferred them to a large petri dish with U-FSW. We scored fertilization success the next morning. Any fertilized eggs were transferred to new petri dishes with U-FSW and maintained daily as previously described. For one genet, embryos developed into larvae, so we prepared a settlement assay in the same manner as was done in 2020 (see below).
Thirty-six to fifty-four hpf, embryos were transferred to clean 4 L plastic buckets filled with filtered seawater and ~10,000 larvae per bucket (Figure S5). We maintained cultures over the next 7-8d by conducting daily 75% water changes using siphons and sieves. Buckets were held within flow-through (183L x 91W x 61H, cm) fiberglass mesocosms ‘raceways’ for temperature regulation. We monitored larval development and behavior until they achieved settlement competency. At first, larvae floated on the surface of the water, followed by elongation and spinning movements. This was then followed by surface swimming. Soon after, larvae dropped down into the water column and once they sank to the bottom of the container and began searching, they were ready to settle. For acroporids in this setting, we have observed time to competency to be 4-10 days post-fertilization.
2.5 Larval settlement
2.5.1 Optimizing set-up
We carried out settlement assays using different techniques in 2020 and 2021. In 2020, we used closed containers (4 L glass aquaria, Aqueon) (Figure 2A) held within partially filled raceways to maintain a stable temperature of ~27-28°C (Figure 2B). We used flat ceramic plugs (~3 cm, white, Boston Aqua Farms) as settlement substrates and covered the entire surface area of the bottom of the tanks (n=72/tank). Plugs were pre-soaked for a few hours before use and maintained on plastic racks (styrene lighting panel) in the tanks. Tanks were filled ~90% with filtered seawater.

Figure 2 Coral larval settlement in closed containers. (A) 4 L glass tanks used for settlement. (B) Settlement tanks are held within partially filled raceways for temperature stability. (C) Naturally occurring crustose coralline algae (CCA) is cultivated within a raceway for using as a settlement cue. (D) Freshly harvested CCA flakes are sprinkled onto a settlement substrate (ceramic plug) to provide chemical cues to larvae. Source: Hanna Koch.
CCA is a known settlement and metamorphosis inducer for coral larvae (Tebben et al., 2015; Elmer et al., 2018; Whitman et al., 2020). As such, it is widely used as a chemical cue in coral settlement studies. Mote’s facility uses natural seawater and thus CCA spp. naturally occur within our system (Figure 2C). Identification of our CCA spp. is currently underway. In 2020, we harvested fresh CCA from an outside raceway, crushed it up using a mortar and pestle, and then sprinkled ~0.04 g of CCA flakes onto each plug (Figure 2D).
In 2021, we optimized our settlement set-up by switching to flow-through containers (Figure 3A). We used 47 L flat-bottomed bins and cut out two 5 cm holes in each of the four sides. The holes were covered with ~100 µm mesh that was affixed using silicone sealant (Dowsil 795). To allow for replicate settlement assays within each bin, we divided them into four quadrants using waterproof corrugated plastic sheets that were secured in place using a hot glue gun. The bins had lips which allowed them to sit in custom-made, collapsible PCV frames. The bins sat in raceways, which were filled with filtered seawater with a flow rate of ~6 L/min (Figure 3B).
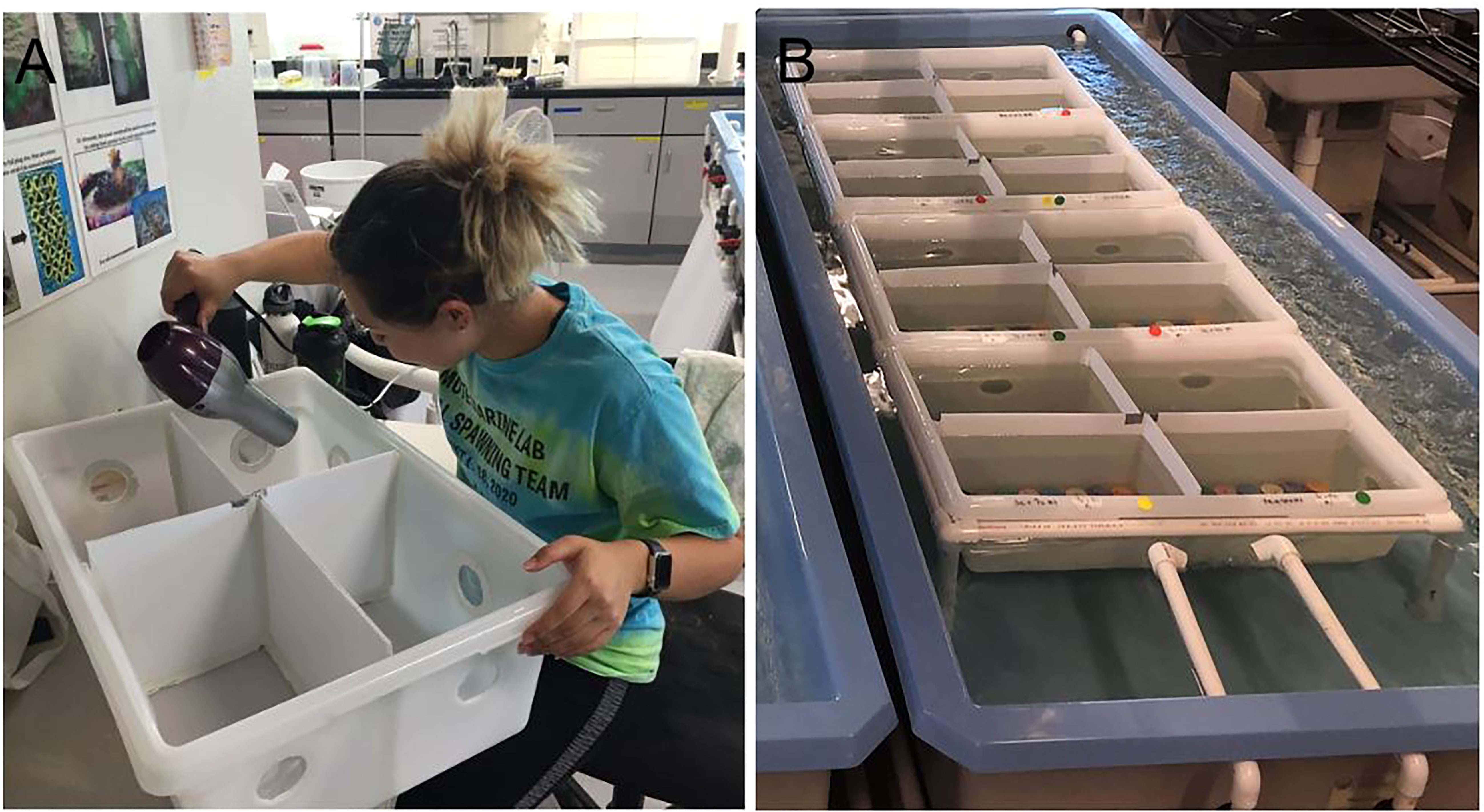
Figure 3 Flow-through settlement containers. (A) To allow seawater to flow through the container, multiple holes are cut out and covered with mesh. To allow for replicate settlement assays, containers were divided into quadrants. (B) Settlement bins are stabilized using a PVC frame and held in a raceway with a continuous flow of fresh seawater. Source: Hanna Koch.
2.5.2 Testing spectral cues
To evaluate larval responses to spectral cues during settlement in 2021, we tested preference for substrate color and used the same type (i.e., shape, material, size) of plugs as previously described but had them made into eight colors (applied/tested by the manufacturer): indigo, white, orange, black, pink, green, purple, and blue. The bins were lined with plugs (n=16/quadrant) (Figure 4A) and held within raceways (Figure 4B). To provide the necessary chemical cue for settlement, we added a standardized amount of CCA to each plug (Figure 4C). To disentangle the response of larvae to the chemical + spectral cues versus the spectral cue alone, we set up replicate control assays (without CCA), which had the same stocking density and settlement duration. For each family (i.e., cross), we conducted replicate (n=2) experimental and control assays. Every plug was labeled using a permanent marker to record the cross on the bottom of the plug, which was then sealed with extra thick gel super glue (Bulk Reef Supply).
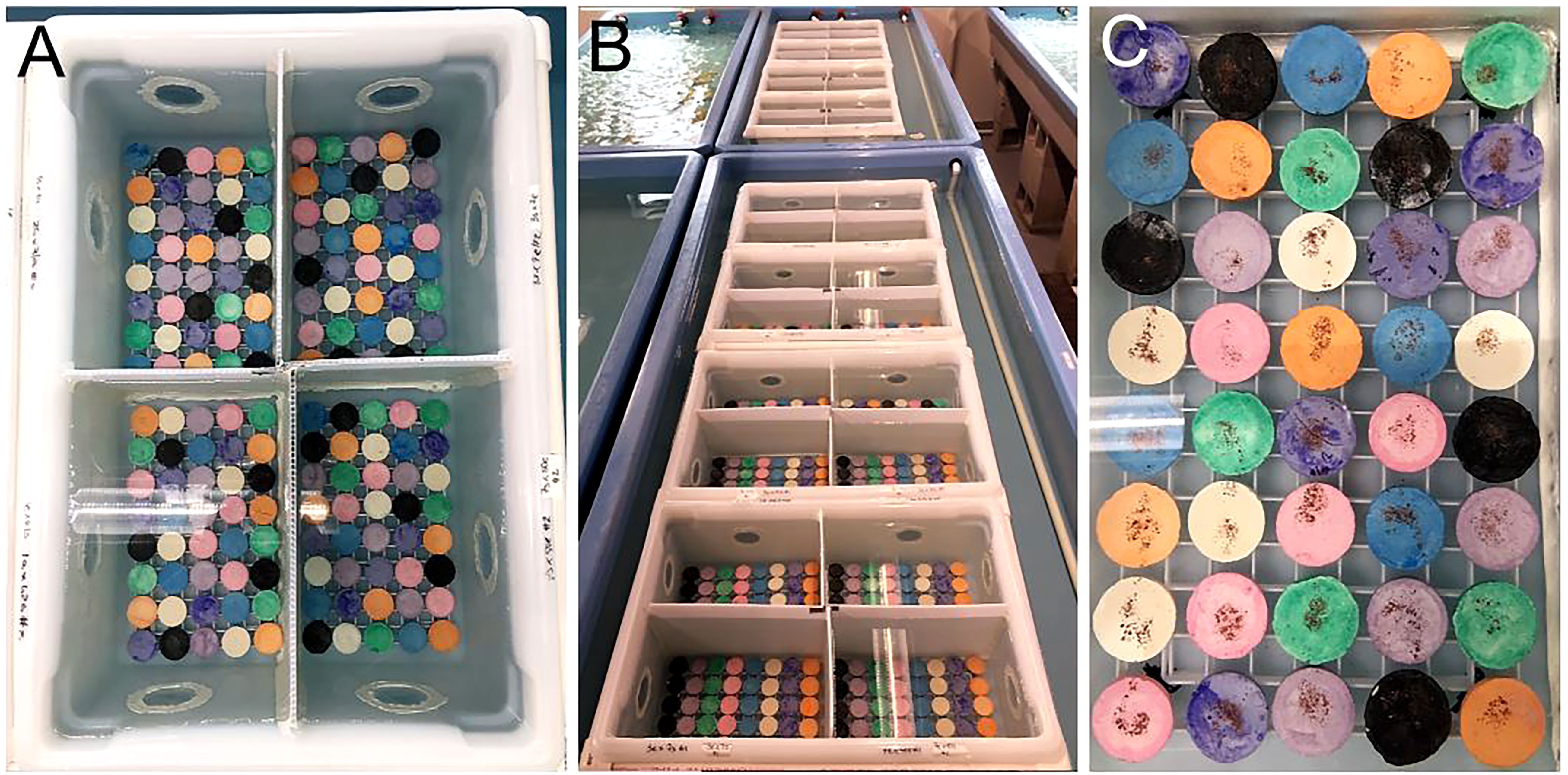
Figure 4 Coral larval settlement in flow-through containers and testing larval preferences associated with spectral cues. (A) Indigo, white, orange, black, pink, green, purple, and blue ceramic substrates (plugs) were used to test which color coral larvae were attracted to during settlement. (B) Settlement assays were conducted in flow-through bins held within raceways with a continuous influx of fresh seawater. (C) CCA flakes were dusted onto each plug to provide a chemical cue for settlement. Source: Hanna Koch.
2.5.3 Settlement
We standardized larval stocking density (1 larva/5mL) (but see below) and settlement duration (3d), which were parameters tested and optimized in previous years (data not included). When we used closed settlement containers in 2020, we conducted daily 75% water changes to maintain optimal water quality. Water changes were not necessary in 2021. After 3d, we removed the plugs, and gently pipetted off any free-standing CCA flakes, which helped to prevent CCA overgrowth of settlers. Plugs were transferred to a new raceway with filtered, aerated ambient seawater (7.8-8 pH, 38 ppt salinity, ~27°C), and received ambient irradiance levels (~10-15 PAR, µmol photons m-2 s-1). The following day, settlers were enumerated using a dissecting microscope and blue light technology (Stereo-Microscope Fluorescence Adapter, RB, Nightsea), which allowed us to use the corals’ natural fluorescence for distinguishing individuals (Figure S6). We also measured settlement densities (number of settlers per plug) and rates (number of settled larvae out of total larvae).
In 2020, during larval rearing, there was a bacterial outbreak in some buckets, which was attributed to overstocking. While we did not observe an increase in mortality, we did notice a reduction in motility which made it difficult to estimate larval stocking densities for such a high volume of larvae during settlement. Therefore, we were not able to standardize stocking densities across the assays or estimate settlement rates. However, settlement still occurred for the majority of families, and in many cases in very high numbers, so we were still able to compare relative settlement success across the different crosses (see Results and Discussion).
In 2021, during larval rearing, we noticed abnormally high larval mortality in all crosses with genet 31 as the dam (i.e., mother/egg donor/’e’). We were thus not able to use the standardized stocking density for the following crosses: 3s x 31e, 7s x 31e, and 31e x 50s. Instead, we settled all available larvae across replicate assays (N=230, 242, and 120, respectively). For this reason, settlement rates of those crosses cannot be directly compared to others (see Results and Discussion).
2.6 Optimizing post-settlement grow-out
After quantifying settlers, empty plugs were removed, and the remaining plugs of each cross were split in half and randomized into two treatments across two raceways based on the presence (‘With’ treatment) -or not (‘Without’ treatment)- of additional lighting and adults (With treatment: 2977 settlers across 465 plugs; Without treatment: 2893 settlers across 464 plugs). For the With treatment, we added 3 Radion XR30 Pro lights (Ecotech Marine) (Figure 5A). Our light regime was based on previous research showing higher early recruit survival under reduced irradiance (Kreh, 2019). Herein, light was measured as the radiant energy between 400 and 700 nm wavelength (i.e., PAR, µmol photons m-2 s-1) as photosynthetic photon flux density (PPFD) (Edmunds et al., 2018), and using a PAR meter (Underwater Quantum Flux, Model MQ-510, Apogee Instruments). Baseline ambient irradiance in the laboratory with only overhead room lights on, was ~14 µmol photons m-2 s-1. Our light regime over the 10-wk post-settlement period was as follows (with changes done incrementally): (1) irradiance increased from ~14 to ~100 µmol photons m-2 s-1; (2) Radion intensity (peak sun) increased from ~25% to ~30%; (3) Point intensity (peak sun) increased from ~75% to ~90%; (4) % blue light increased from 0 to 10; (5) % violet, green, red, warm, cool and UV light remained consistent at 37, 61, 61, 66, 71, and 73, respectively. The Without treatment only received overhead laboratory lighting (Figure 5A), which had a stable irradiance of ~14 µmol photons m-2 s-1. All lights were on a similar 12hr day/night schedule.
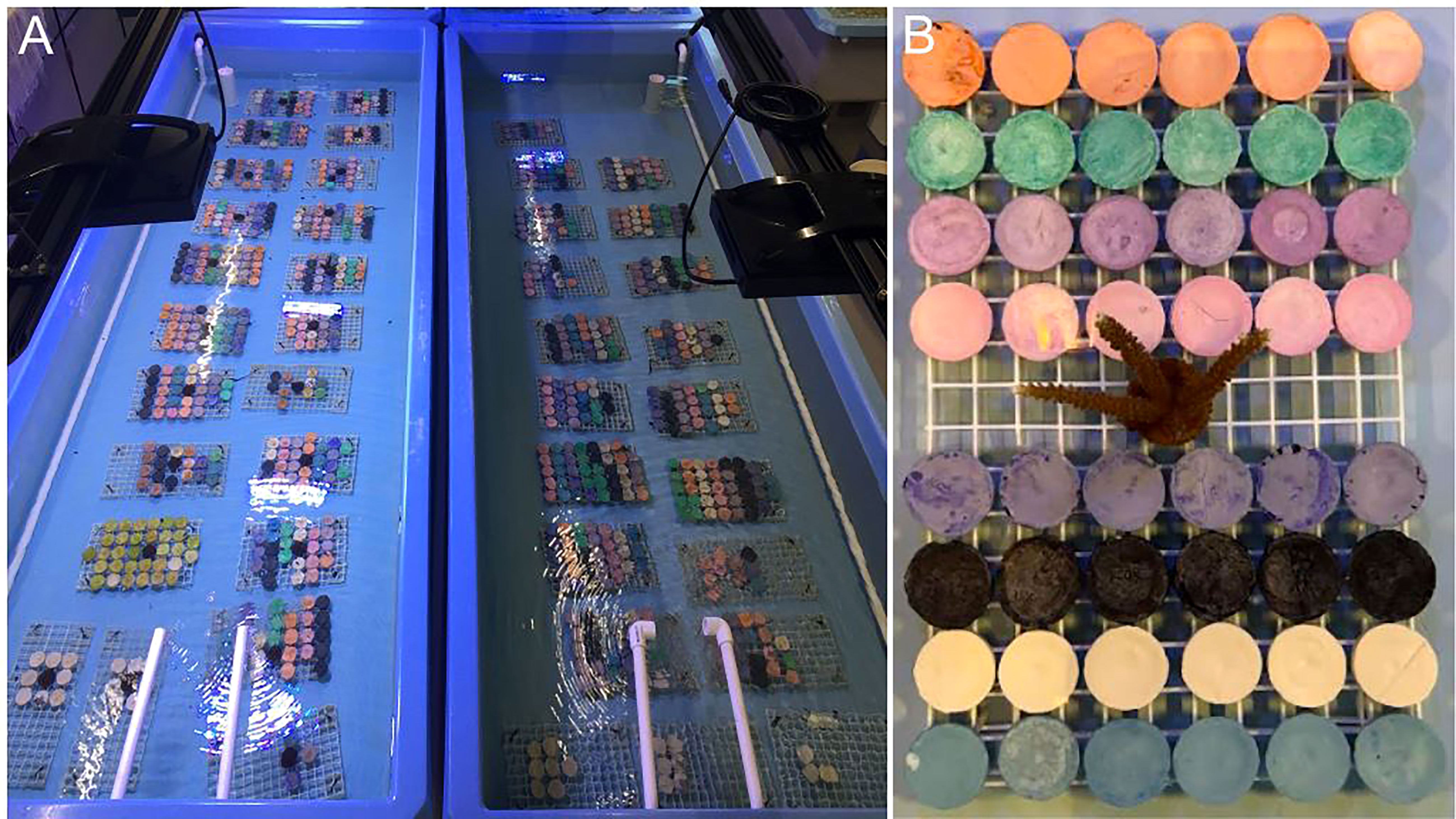
Figure 5 Optimizing grow-out of sexual recruits. (A) Crosses were equally divided into two treatments based on additional lighting (With treatment, left) or only ambient laboratory lighting (Without treatment, right). (B) Every rack of corals in the With treatment also received one adult coral to encourage faster uptake of algal symbionts. Source: Hanna Koch.
Every rack of corals in the With treatment received 1 adult A. cervicornis coral (Figure 5B), which was given a short Lugol’s bath (Lugol’s Iodine) prior to addition. Adult corals were 2-yr old plugs that had remained in Mote’s ex-situ nursery for the duration of their life. To monitor survivorship and zooxanthellae acquisition over time, every plug with sexual recruits was inspected under a dissecting microscope on a weekly basis, for 10 weeks, to record if there were living recruits present and if so, if any had symbionts. The plug was the unit of measure and given a 0 or 1 for both metrics. At the end of the 10-wk study, ten plugs per family and treatment were chosen at random for the final growth comparison. Images of plugs were taken in a standardized manner using a camera (Olympus TG-6) to capture images from a fixed distance. A ruler was used for scale. Using ImageJ, the outline of every recruit on each plug was traced to extract surface area measurements. Corals and raceways were maintained throughout the study using the same husbandry regime (see below).
2.7 Coral husbandry
We developed a standardized raceway maintenance and coral husbandry protocol that was utilized for both studies in 2020 and 2021, and which promotes coral growth and survival by providing a healthy environment with stable, optimal conditions. The elements of this regime included biological control (i.e., herbivorous invertebrate grazers), daily feeding, daily siphoning and basting, bi-weekly raceway cleaning, optimal flow rates and water quality. To mitigate algal fouling, we deployed to each raceway ~1000 Batillaria spp., which are small intertidal snails that are effective grazers and do not irritate small, delicate recruits. We have never observed recruit mortality as a result of snail grazing using this species. All snails were quarantined for one week and washed using a 3% H2O2solution prior to use. We fed recruits a daily diet of 57 g of Golden Pearls (5-50 µm, Aquatic Foods Inc.), 57 g Reef-Roids (Polyp Lab), and a 10 mL solution of Microvore, Microblast and Zooplankton-S (Brightwell Aquatics). We turned the air and water pumps off before broadcast feeding across the raceway, making sure to target each rack of corals. They were allowed to feed for 30min, after which time, we turned the pumps back on. Also on a daily basis, we siphoned every raceway to remove snail detritus and prevent algal blooms. We also gently basted every plug using a turkey baster to remove nuisance microorganisms. Over time, more algae grew in raceways than snails could control, so we acid washed raceways on a bi-weekly basis. We used 50% muriatic acid and a non-scratch sponge to clean them, followed by freshwater and seawater rinses. Immediately after, we filled it back up again. Corals were temporarily (<1hr) moved to another raceway with identical conditions, and then moved back. Flow rates were maintained around 6L/min, and optimal water quality parameters included: ~25-27°C, 7.8-8 pH, and 36-38 ppt salinity.
2.8 Statistical analyses
We performed all statistical analyses in R v.4.0.3 (R Core Team, 2020). Data were checked for normality using the Shapiro–Wilk test and for equality of variance using Levene’s test or the Fligner-Killeen test if data were non-normal. To test for significant differences among groups, non-parametric tests (e.g., Kruskall-Wallis, Wilcoxon) or mixed-effects models (e.g., LMM) were used when data violated assumptions of normality. Following the use of omnibus tests, we then ran matching post-hoc tests (e.g., Dunn test, non-parametric) to look for significant pairwise differences. All post-hoc tests were run with a Bonferroni correction factor to account for multiple testing. These were the set of tests used for testing for significant differences in: (1) fertilization rates, (2) settlement rates, (3) settlement densities, and (4) recruit size. For optimizing grow-out of sexual recruits and comparing treatments, survivorship was analyzed using a Kaplan-Meier survival curve and median survival times of treatments were compared using a log rank test (X2). To test the effects of treatment, time and their interaction on zooxanthellae acquisition, we used a linear mixed-effects model (LMM: lme4 package) with treatment and time as fixed effects and time nested within replicate plug nested within treatment as random effects to account for temporal pseudo-replication. We conducted model fitting and used Akaike information criterion (AIC) to select the best fit model of our data.
2.9 Active restoration
Once recruits from the 2020 cohort reached ~2 ½ cm in diameter, we carried out two different strategies for outplanting on degraded reefs in the Lower Florida Keys. For the first strategy, we outplanted subsets of single plugs where each plug represented a novel genet. The second strategy involved fragmenting each novel genet into replicate clonal fragments, and then outplanting them as a cluster (Figure 6A). For outplanting singles, we removed the plug stems using a bone cutter, and then epoxied each plug to bare reef using 2-part epoxy and in a 10x10m grid fashion for monitoring purposes (5 corals per 2m2). For the clusters, we used a wet C40 diamond band saw (Gryphon Corporation, Sylmar, CA, US) to cut corals into five similarly sized fragments. Fragments were grown out for another few months in an outdoor raceway in Mote’s land-based nursery under standardized conditions (i.e., ~25-27°C, 7.8-8 pH, and 36-38 ppt salinity) and with natural light cycles. The daily husbandry regime was the same as previously described. Once fragments were full-grown, the clonal sets were outplanted in small clusters and affixed to the reef using the same attachment method as the single plugs. Each cluster represented a single genet and was tagged for monitoring purposes (Figure 6B). Each genet was fragmented and outplanted only once. For both strategies, corals from the different families were randomized to ensure a diversity of genets and families at each outplant site. We also targeted a diversity of reefs and habitat types (see Table 2), making sure to only outplant in this species’ natural habitat. All corals were healthy and required to pass a certified vet check prior to outplanting. We conducted 1-month monitoring of outplants on SCUBA to record coral and genet survivorship.
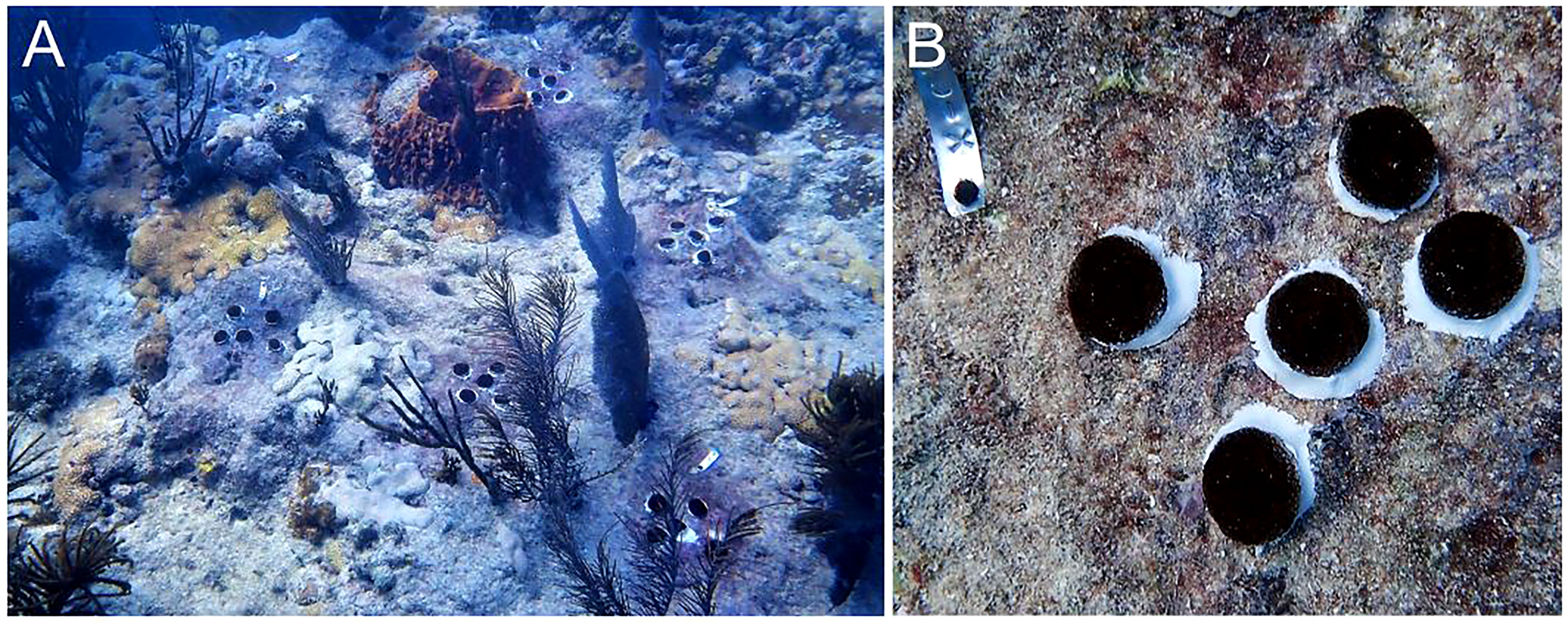
Figure 6 Active restoration using sexual recruits produced via ASR. (A) Outplant event where each cluster of corals represents a different genet from the same or different family. (B) One method of outplanting involves fragmenting a single genet into five clonal replicates, which are then outplanted as a cluster in order to form (via fusion) a larger colony faster; every outplant or cluster is tagged for monitoring purposes. Source: Erich Bartels.
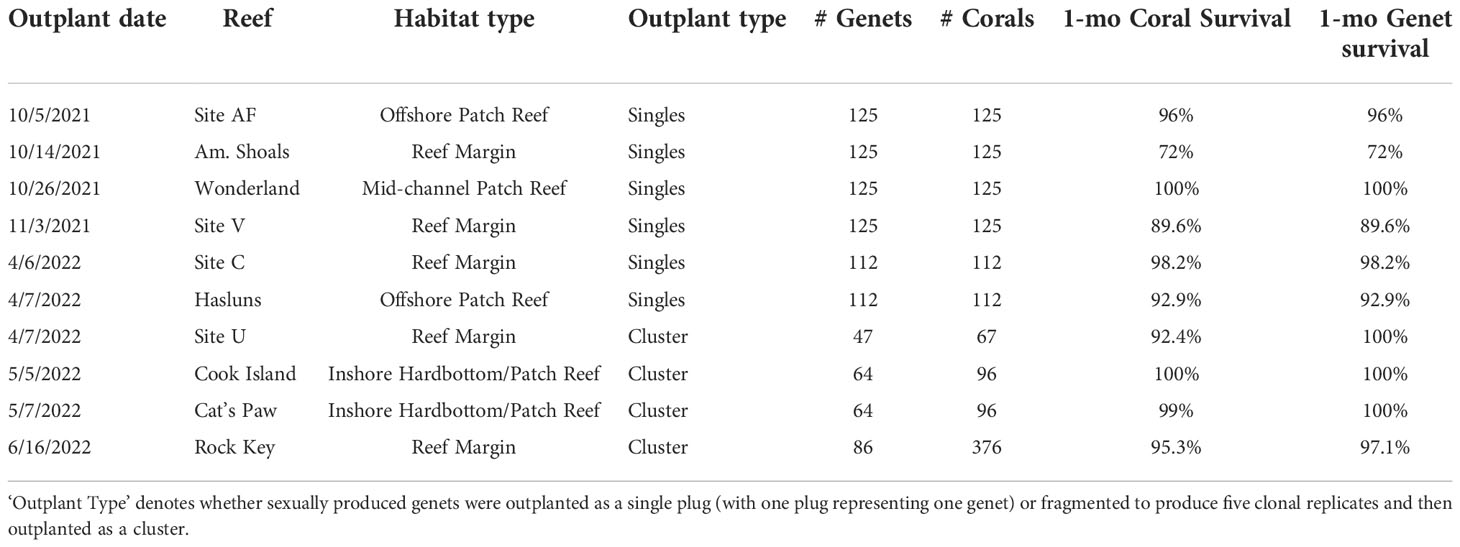
Table 2 Outplant and survival data for A. cervicornis sexual recruits produced via ASR in 2020 for active restoration purposes on Florida’s Coral Reef.
3 Results
3.1 Spawning nurseries
The use of offshore spawning nurseries worked well for creating reliable access to sexually mature corals that could be easily transported between the field and laboratory for annual ex-situ ASR efforts. A couple of minor complications arose from the occurrence of storms. In 2020, the genet 31 tree in the Sand Key nursery went missing after a tropical storm. In 2021, colonies of genet 13 in the Looe Key nursery also went missing after turbulent weather. In order to maintain colony and nursery health, some colonies, or portions of colonies, were culled if tissue loss or excessive fouling was observed. Having nursery redundancy was helpful. It prevented the loss of genotypic diversity, especially in cases where entire trees in one nursery were lost due to inclement weather.
3.2 Morphometric and sexual maturity assessments
Having sampled colonies that were above the predicted puberty size (~50-100 x102 cm3) and apparently healthy, the results are as follows (Table S1). In 2020, the mean colony size (volume) of Sand Key corals (N=129) and genets (N=11) was ~266 x102 cm3, and 49/61 sampled corals (80%) were gravid. Every genet had gravid colonies except 41. For genet 41, colony sizes ranged from 68 to 234 x102 cm3, with a mean volume ± SEM (standard error of the mean) of 139.77 ± 15.39 x102 cm3, which was the smallest relative to all other genets. Because not every branch may develop gametes -and smaller colonies may have fewer large branches- it is possible a colony is indeed gravid, but our sampling did not capture it. Nonetheless, one does not want to sample in a way that compromises the integrity of the entire colony. Furthermore, we were only permitted to sample up to three branches per colony. In 2021, the mean colony size of Looe Key corals (N=39) and genets (N=9) was ~684 x102 cm3 and 39/39 (100%) were gravid.
3.3 Ex-situ spawning
Across years, genets, and ramets (clonal colonies) -and in terms of nights after the full moon (NAFM) and minutes after sunset (MAS)- corals spawned synchronously overall (Figure 7; Table 1). In both years, every colony and genet spawned, with nearly all colonies spawning across multiple nights, often consecutively. These data are consistent with predicted spawning times based on historical observations for this species, which is 1-15 NAFM with peak spawning activity around 3-6 NAFM (Jordan, 2018). Peaks refer to periods when the highest degree of spawning activity is observed, which was night 6 in 2020 (33/52 colonies and 8/10 genets) and night 7 in 2021 (17/17 colonies and 9/9 genets). Fewer ramets were brought in for ex-situ spawning in 2021 as a result of their increased size, which limited the number of colonies that could fit in the mesocosms. 2021 was considered a split spawn year as corals spawned a month earlier than predicted (i.e., after the July full moon).
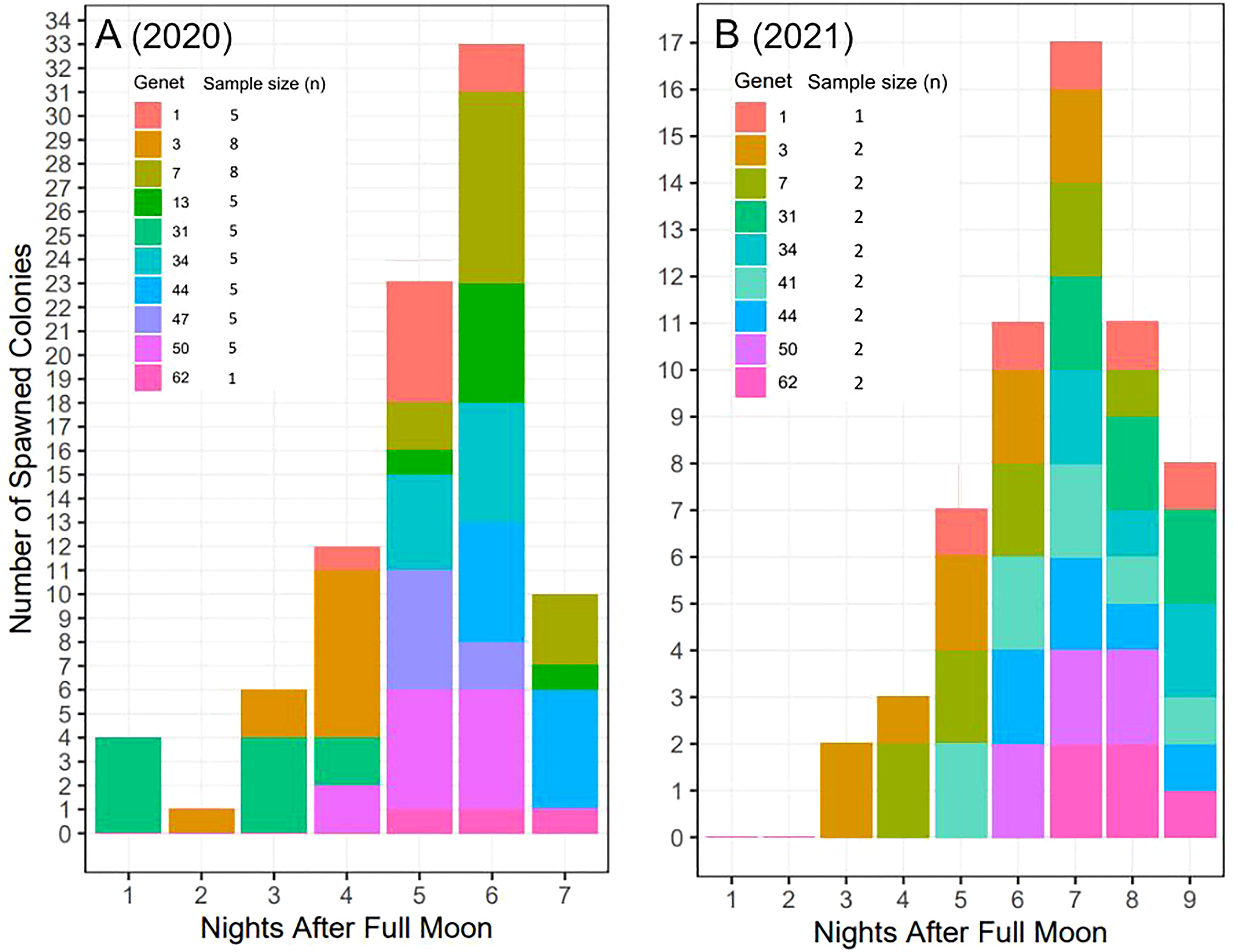
Figure 7 Ex-situ broadcast spawning timing data for A. cervicornis genets used for managed breeding and active restoration. Timing is relative to the (A) August 3, 2020 full moon (52 colonies; 10 genets) and (B) July 23, 2021 full moon (17 colonies; 9 genets). In both years, every colony and genet spawned, with nearly all colonies spawning across multiple nights, often consecutively. Across both years, corals spawned during the predicted window for this species and region (i.e., 1-15 NAFM), with peak spawning activity observed on night 6 in 2020 and on night 7 in 2021.
Some genet-specific spawning patterns in terms of NAFM emerged from this data (Figure 7). For example, across both years, genets 1 and 50 spawned in the middle of the observed window, genet 3 spawned early, and genet 62 spawned late in the window. Alternatively, differences between the two years were observed for certain genets. For example, genet 31 spawned in the first half of the window in 2020 and then in the last half of the window in 2021. Similarly, genet 7 spawned later in 2020 and then earlier in 2021. Interestingly, in 2020, genet 31 was held in the same mesocosm as genet 3, while in 2021, genet 7 was held with genet 3.
According to historical observations from the field of broadcast spawning times for this species and region (Marhaver et al., 2017a; Jordan, 2018), the predicted spawning window in terms of minutes after sunset (MAS) is 125-200. Across seven consecutive nights (1-7 NAFM) in 2020, all corals started spawning within 42 min of each other (154-196 MAS) and ended spawning within 50 min of each other (189-239 MAS) (Table 1). Across 7 consecutive nights (3-9 NAFM) in 2021, all corals started spawning within 48 min of each other (157-205 MAS) and ended spawning within 45 min of each other (184-229 MAS) (Table 1). Across both years, all corals started spawning within 51 min of each other (154-205 MAS), stopped spawning within 55 min of each other (184-239 MAS), and had a full spawning window of 154-239 MAS. Thus, comparing the predicted full window (125-200 MAS) to the observed full window (154-239 MAS), we can say our corals spawned within minutes of the predictions, indicating high spawning synchrony and predictability for our nursery corals.
3.4 Assisted fertilization
3.4.1 Experimental crosses
Across both years, overall fertilization success was ~95% (Figure 8). In 2020, there were significant differences in fertilization success across the 29 two-parent crosses (Kruskal-Wallis: X2 = 177.3, d.f. = 28, p< 0.0001), but no significant differences were found between the 12 pairs of direct and reciprocal crosses (p-adj. > 0.05, post-hoc Dunn test with Bonferroni correction factor) (Figure 8A). Only one pair of crosses was not compatible (34 x 47), which was incompatible in both directions and reproducible across multiple nights (34e x 47s: 0.04% on 8/8/20 and 0.01% on 8/9/20; 34s x 47e: 0.05% on 8/8/2020 and 0.02% on 8/9/2020). Excluding this cross, overall fertilization success was ~96% in 2020. Similarly in 2021, there were significant differences in fertilization success across the 20 two-parent crosses (Kruskal-Wallis: X2 = 83.14, d.f. = 19, p< 0.0001), but no significant differences were found between the 8 pairs of direct and reciprocal crosses (p-adj > 0.05, post-hoc Dunn test with Bonferroni correction factor) (Figure 8B). Overall fertilization success was ~95% in 2021.
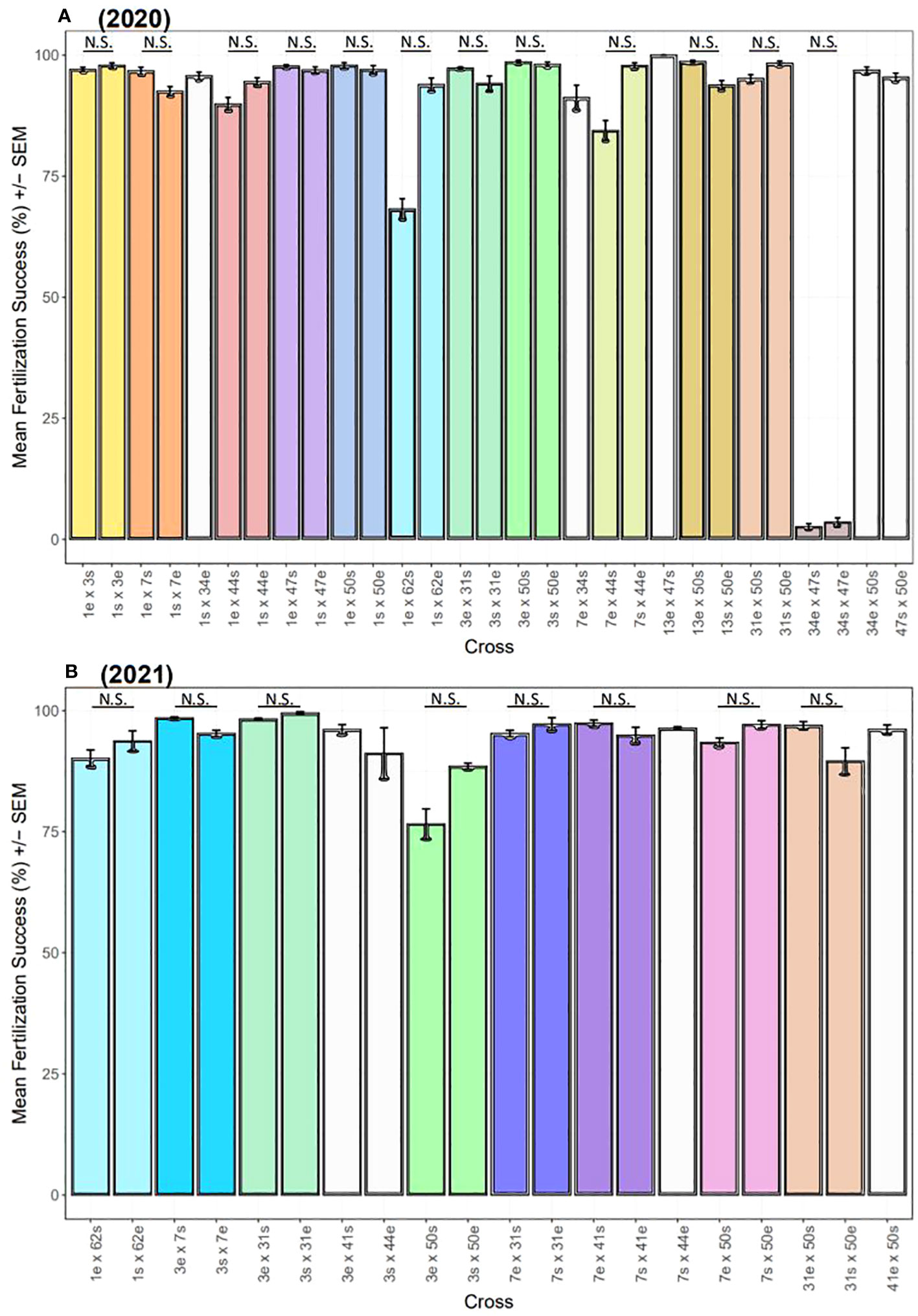
Figure 8 Fertilization success of two-parent A. cervicornis controlled crosses across two years. Dams (mothers) and sires (fathers) are indicated by ‘e’ (egg donor) and ‘s’ (sperm donor), respectively. (A) In 2020, there were significant differences in fertilization success (mean % ± SEM) across the 29 two-parent crosses (statistics in text), but no significant differences (‘N.S.’) were found between the 12 pairs of direct and reciprocal crosses. Excluding 34 x 47, overall fertilization success was ~96%. (B) In 2021, there were significant differences in fertilization success (mean % ± SEM) across the 20 two-parent crosses (statistics in text), but no significant differences (‘N.S.’) were found between the 8 pairs of direct and reciprocal crosses. Overall fertilization success was ~95%. In both plots, direct and reciprocal crosses are paired by color, uni-directional crosses are white, and the 2020 crosses repeated in 2021 have the same color scheme.
3.4.2 Selfing controls
We observed a small degree of selfing for some genets (Figure S7). For the No Sperm controls, fertilized eggs were observed for genets 31, 44, and 50 (Figure S7A). There were significant differences in mean fertilization success across genets (Kruskal-Wallis: X2 = 42.5, d.f. = 8, p< 0.0001), with genet 44 having the most embryos, followed by genets 50 and 31. Within one week, all embryos had perished. For the Sperm + Egg controls, fertilized eggs were observed for all genets except 1, 7, and 50 (Figure S7B). There were no significant differences in mean fertilization success across genets (Kruskal-Wallis: X2 = 8.46, d.f. = 8, p = 0.4). Within one week, all embryos had perished, except for genet 44, which had three larvae develop. We prepared a settlement assay for these few larvae, using standardized conditions, but none of them settled and they too perished shortly thereafter.
3.5 Larval settlement
3.5.1 Settlement by family
Overall, we observed differences in settlement success by cross (Figure 9). In 2020, some crosses yielded no settlers, and in some cases, settlement only occurred in one direction of the cross (Figure 9A). For example, crosses 13e x 47s and 47s x 50e yielded no settlers. Crosses 1e x 47s, 1s x 50e, 1e x 62s, 3s x 31e, 13s x 50e, and 31e x 50s also produced no settlers but settlers were produced in the reciprocal of those crosses. Furthermore, 3e x 50s and 7s x 44e produced almost no settlers (1 and 5, respectively) while the reciprocals had hundreds of settlers. Fertilization success was near zero for 34 x 47, and no larvae or settlers were produced from either direction of the cross, indicating complete incompatibility. Crosses 1e x 3s and 7e x 44s produced the most settlers (>800). The disparate outcome of 13e x 50s compared to 13s x 50e was a reproducible result. In 2019, genets 13 and 50 were fertilized and settled under previously described standardized conditions. Fertilization and settlement results across the two years were as follows. Fertilization (2019/2020: 13e x 50s = 80%/99%; 13s x 50e: 50%/94%); Number of settlers produced (2019/2020: 13e x 50s = 195/198; 13s x 50e: 4/0); Number of surviving recruits 3-mo post-settlement (2019/2020: 13e x 50s = 152/194; 13s x 50e: 0/0).
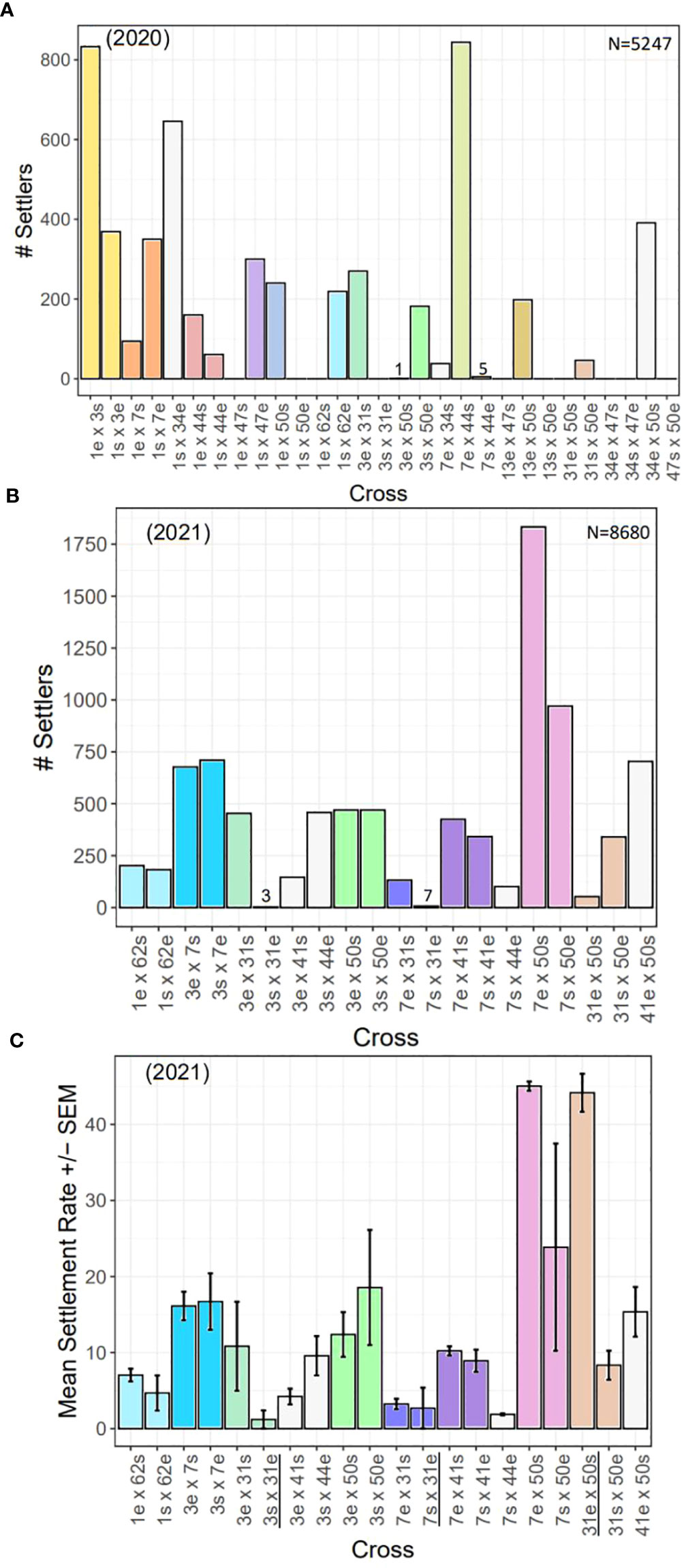
Figure 9 Settlement by year and cross. (A) Total number of settlers for each cross conducted in 2020 (N=5247 settlers). (B) Total number of settlers for each cross conducted in 2021 (N=8680 settlers). (C) Mean settlement rates (± SEM) for each cross in 2021. There were significant differences in settlement rates across families (statistics in text). All settlement assays had the same larval stocking density, except those with 31e (black underlined, see Methods & Results), so they cannot be directly compared. In all plots, direct and reciprocal crosses are paired by color, uni-directional crosses are white, and the 2020 crosses repeated in 2021 have the same color scheme.
In 2021, when larval stocking densities were standardized, we found more consistent results, in general, in terms of total number of settlers between direct and reciprocal crosses (Figure 9B). For example, 1 x 62, 3 x 7, 3 x 50, and 7 x 41 all yielded a similar number of settlers in both directions of the cross. However, we did find four cases where one direction of the cross was more successful than its reciprocal (i.e., 3 x 31, 7 x 31, 7 x 50, and 31 x 50). Although, in the case of 7 x 50, while one direction of the cross yielded nearly twice as many settlers as its reciprocal, the reciprocal still had the second highest number of settlers overall. Thus, the most successful crosses were 7 x 50, followed by 3 x 7, while the least successful crosses were 7 x 44, and any cross with 31e.
We compared settlement rates across families for the 2021 cohort (Figure 9C), and found signficant differences (Kruskal-Wallis: X2 = 33.07, d.f. = 19, p< 0.05). However, high variability in the data and low replication likely attributed to not being able to detect significant pairwise differences between direct and reciprocal crosses (p-adj. > 0.05, post hoc Dunn test with Bonferonni correction factor). Nonetheless, the most successful cross with the highest mean settlement rate of ~45% was 7e x 50s. Its reciprocal (7s x 50e) had the next highest mean settlement rate (~25%). Eight crosses had mean settlement rates of ~10-20%, while another seven had less than 10%. Settlement rates for crosses with 31e cannot be directly compared as they were settled with different densities (see Methods and paragraghs below).
To test the reproducibility of our results, we repeated certain failed 2020 crosses in 2021. These included 1e x 62s, 3s x 31e, 3e x 50s, 7s x 44e, and 31e x 50s (Figures 9A, B). Contrary to our 2020 results, we achieved settlement for 1e x 62s and 3e x 50s in 2021 (Figure 9B). In 2021, 7s x 44e had among the lowest mean settlement rates, but ~100 total settlers were produced from this cross, which suggests this cross is indeed viable. However, similar to our 2020 results, all crosses with 31e were the least successful (Figure 9B). In 2020, all crosses with 31e failed to produce settlers, while in 2021 the total number of settlers of such crosses were as follows: 3s x 31e (n=3); 7s x 31e (n=7); 31e x 50s (n=53). By three months post-settlement, the number of surviving recruits of each cross were as follows: 3s x 31e (n=0); 7s x 31e (n=1); 31e x 50s (n=19).
During larval rearing, we observed anomalously high larval mortality in cultures containing 31e. When it came time for settlement, we enumerated only ~200 remaining larvae for each cross (Figure 10A). The number of larvae produced is a product of many factors including how many gamete bundles are collected, and eggs fertilized, which are not necessarily standardized during mass production, but we would expect that if a cross had high fertilization -and was viable- then we would retain at least as many larvae as embryos were counted when scoring fertilization success, which is only a small subset of total embryos produced. However, when comparing the ratio of larvae produced to embryos counted for each cross, we found that crosses with 31e had anywhere from 4 to 9 times more embryos than larvae (Figure 10B), indicating that most of the fertilized eggs had perished. To assess the general developmental state, morphology and behavior of the remaining larvae for each cross, we investigated a subsample under a dissecting microscope and found that many of them did not have the typical shape (Figure 10C) and swimming behavior as expected, but instead were mal/deformed, stunted, and spinning in place (Figure 10D).
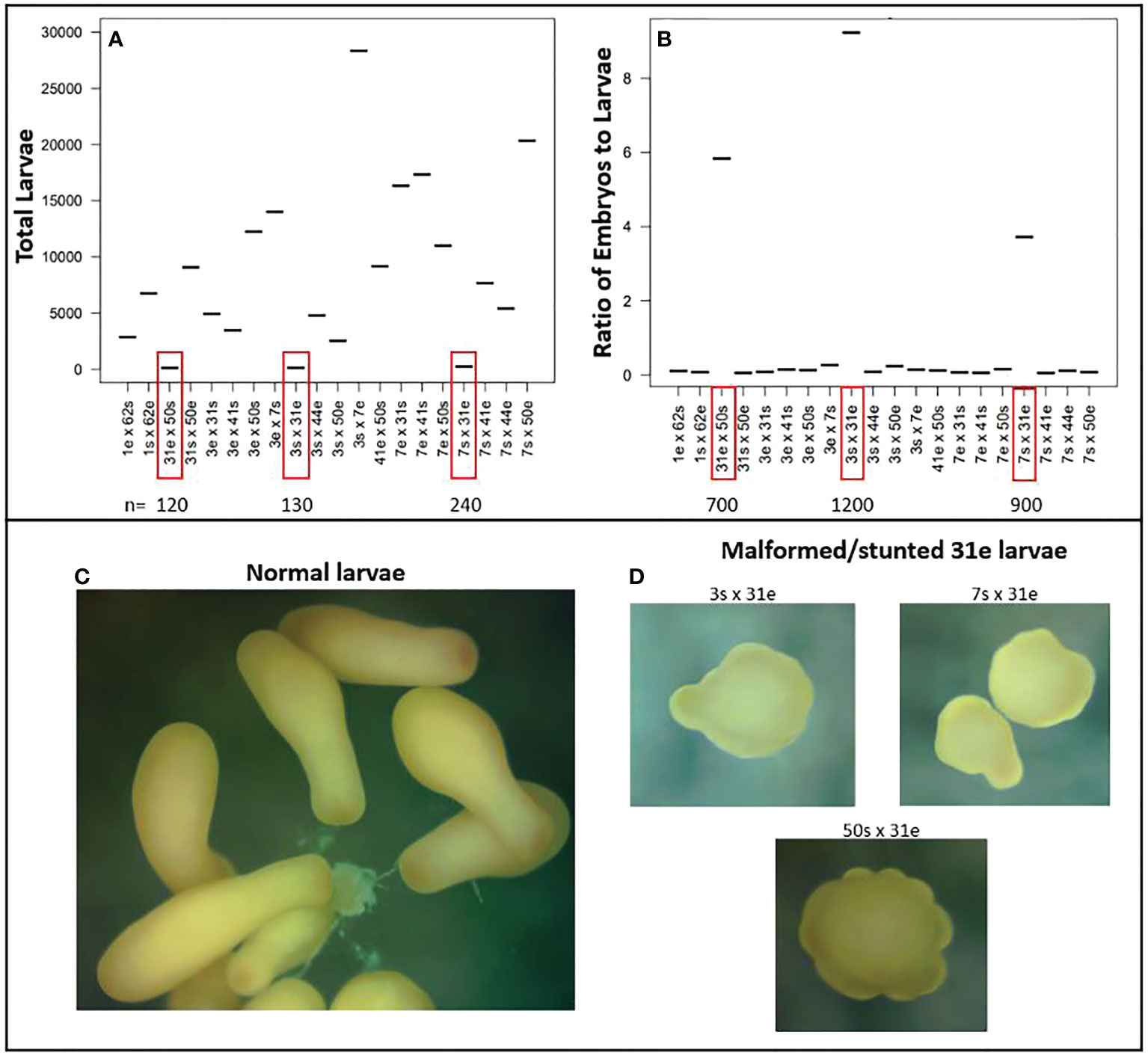
Figure 10 Unsuccessful crosses with genet 31 as the dam (egg donor). (A) Total number of larvae produced for each cross. Crosses with 31e had high larval mortality and low final yield. (B) Ratio of enumerated fertilized eggs to total larvae produced for each cross. Crosses with 31e had anywhere from 4-9x more embryos than larvae, again indicating high larval mortality. (C) The typical bowling pin shape of a competent planula larva. (D) Abnormal morphology observed for subsamples of larvae from crosses with 31e.
3.5.2 Testing spectral cues
We evaluated larval responses to spectral cues to test whether substrate color could be used to increase settlement (Figure 11). While larvae settled on plugs of all colors, we found significant differences in settlement density (Kruskal-Wallis: X2 = 165.2, d.f. = 7, p< 0.0001), with larvae preferring pink and purple plugs significantly more (p-adj.< 0.05; Dunn test with Bonferroni correction factor) (Figure 11A), which was consistent with our prediction that larvae would prefer substrates similar in color to CCA. The least preferred color was green, followed by blue and orange. To disentangle responses to chemical versus spectral cues of CCA, we conducted control assays without CCA and found significant differences across groups (Kruskal-Wallis: X2 = 43, d.f. = 7, p< 0.0001), with larvae settling in significantly higher densities on white plugs (p-adj.< 0.05; Dunn test with Bonferroni correction factor) (Figure 11B). The colors with the next highest values for median settlement density in the controls were pink and purple.
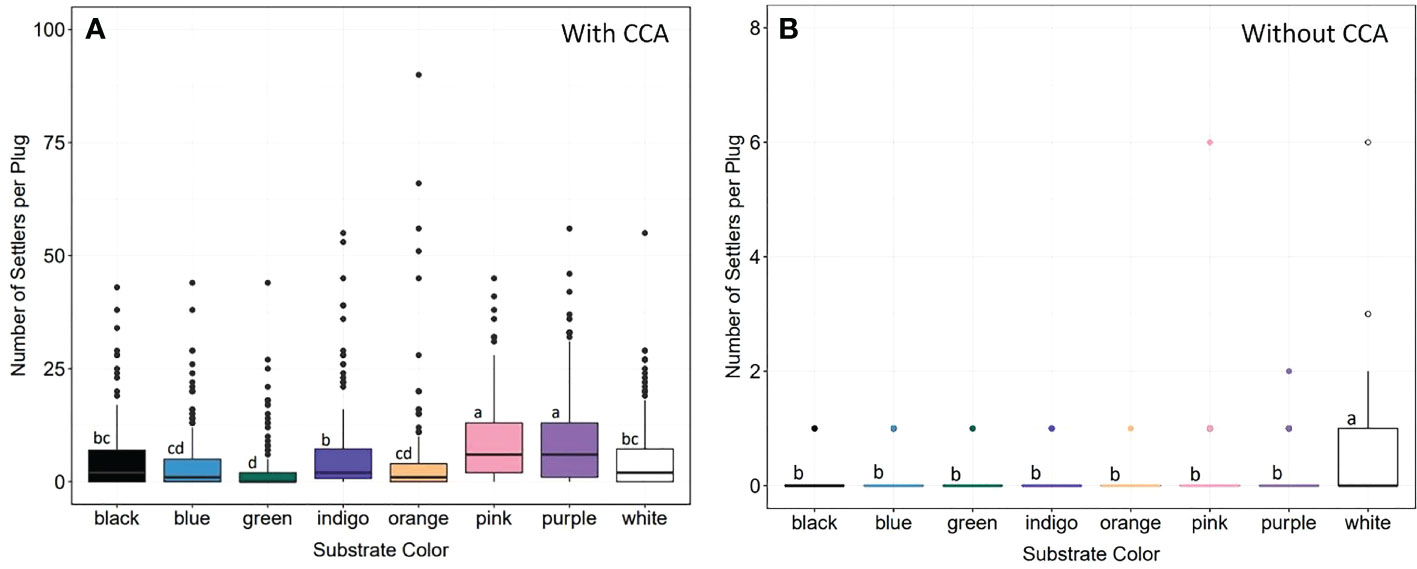
Figure 11 Larval settlement preferences and spectral cues. (A) Settlement density on eight different colored substrates with CCA. Larvae settled on all colors, but in significantly higher densities on pink and purple substrates (statistics in text). Green was the least popular, followed by blue and orange. (B) Settlement density on differently colored substrates without CCA. There were significant differences across groups (statistics in text), but larvae preferred white substrates. Letters denote significant differences between groups (Dunn test with Bonferroni correction factor).
3.6 Optimizing post-settlement grow-out
To test the impact of additional lighting and the presence of adult corals with established algal symbiosis on the survival, growth and uptake of zooxanthellae of recruits, we reared them with and without these two factors for nearly three months (Figure 12). Overall, survivorship in both treatments remained high by the end of the study (>96%, Kaplan-Meier Survival Curve), and although median survival time was slightly higher in the With treatment, it was not significantly so (Log-rank test: X2 = 0.6, d.f. = 1, p = 0.4) (Figure 12A). However, when comparing the proportion of plugs with recruits infected with zooxanthellae, we found a significant interaction between treatment and time (LMM: X2 = 289.45, d.f. = 10, p<0.0001) indicating that there are significant differences between our treatments and over time (Figure 12B). It therefore appears that the uptake of algal symbionts occurred faster in the presence of additional lighting and adult corals (With treatment). This finding is corroborated by our photo-tracking of a subset of recruits in the different treatments over time where it appears that symbionts can be seen occurring earlier in the recruit in the With treatment (Figure S8). Although, this is only one example. We also found a significant difference in the median surface area of recruits in the With treatment (Wilcoxon: W=44514, p< 0.0001), indicating that under additional lighting, recruits grew faster and were larger by the end of the study (Figure 12C).
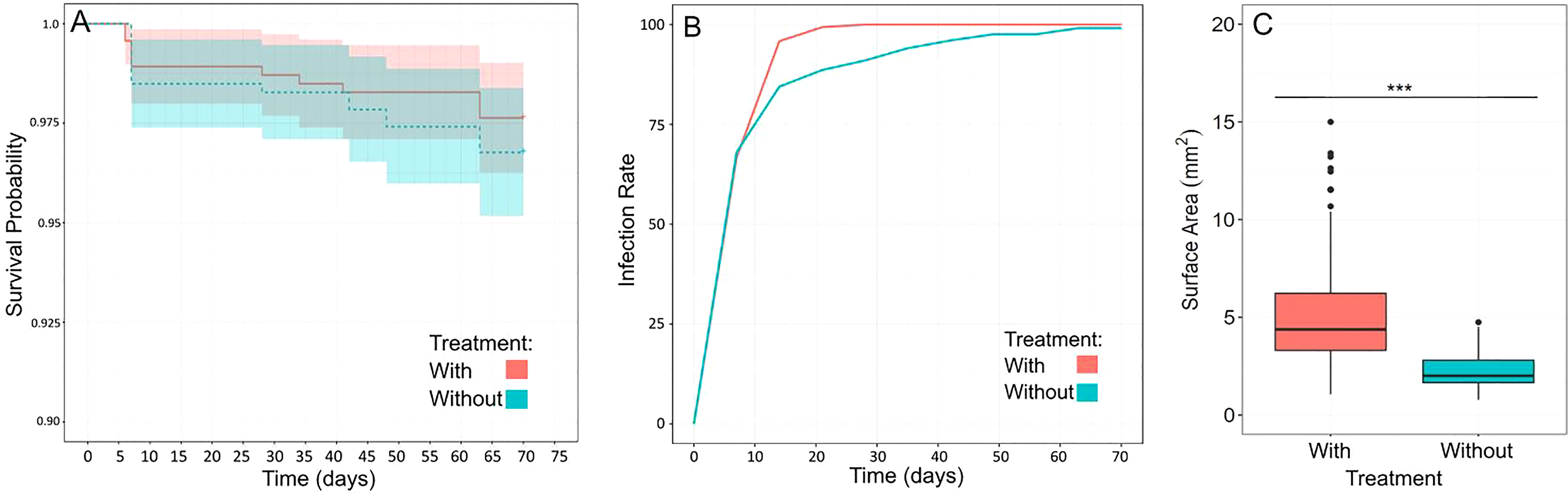
Figure 12 Grow-out of recruits in the absence (Without treatment) or presence (With treatment) of additional lighting and adult corals. (A) Survival probability curve for the two treatments showing no significant difference in median survival times (statistics in text). While survival in the With treatment was slightly higher, it remained high in both treatments (>96%) by the end of the study. (B) Proportion of plugs with recruits infected with algal symbionts over time in the different treatments. There was a significant interaction between treatment and time indicating symbiont uptake occurred more quickly over time in the With treatment (statistics in text). (C) Size (median surface area) of a subset of recruits from both treatments. Recruits in the With treatment were significantly larger (indicated by asterisks) by the end of the study (statistics in text).
3.7 Active restoration
To increase the adaptive potential of restored populations, one strategy is to outplant a diversity of sexually produced corals, which we did here using the 2020 cohort. Overall, we outplanted 1,359 corals representing 19 different families and 985 novel genets across ten different reefs (Table 2). Overall mean coral and genet survival was 94% and 95%, respectively (Table 2). For single plugs, coral survival equates to genet survival, which was 91% on average (Table 2). Mean coral and genet survival for the clusters was ~97% and 99%, respectively (Table 2). Even without knowing the source(s) of mortality, these initial results show that with single plug outplants, coral loss equates to genet loss, whereas with the clusters, even if some replicate fragments within a cluster perish, the genet is not necessarily lost.
4 Discussion
The purpose of this study was to combine basic and applied research questions for optimizing ASR techniques to upscale the number and diversity of corals used for actively restoring Florida’s Coral Reef. There are several components to the ASR process and herein we focused on better understanding broodstock compatibility in terms of spawning synchrony and fertilization success, testing spectral cues associated with substrate color to enhance settlement, and optimizing the grow-out of sexual recruits to reduce the time from production to restoration. We provide a discussion of our results as well as recommendations for those interested in conducting ASR.
4.1 Spawning
Having reliable and easy access to sexually mature corals is important for ASR but not always attainable, especially in areas where natural populations are severely degraded (e.g., Florida). The use of in-situ spawning nurseries has several advantages and can be created for any coral species (Amar and Rinkevich, 2007). Depending on site location, conditions can promote growth which can increase the number of propagules harvested for ASR since fecundity is often positively correlated with colony size (Nozawa and Lin, 2014; Foster and Gilmour, 2020). Special aquaria can be used to induce corals to spawn ex-situ (Craggs et al., 2017), but their capacity to hold many and large corals is limited, so maintaining corals in a field nursery allows for upscaling and ensuring access to environmental cues required for gametogenesis. Field nurseries are more likely to be shielded from light pollution as well, which can disrupt the sexual cycle (Ayalon et al., 2020). Corals in a mid-water floating nursery may also be more protected from impacts like predation, sedimentation, and destructive human activities. It is possible to harvest gametes from within the spawning nursery, but fieldwork logistics are more complicated at night, especially if weather conditions are not ideal and nurseries are not located close to the laboratory. Thus, we find it is easier and safer to bring the corals to the laboratory a few days before the full moon (Figure 1).
This strategy does not appear to negatively impact the corals’ spawning timing as all corals and genets spawned within predicted timeframes regarding NAFM and MAS (Figure 8; Table 1). Ultimately, predictability and synchronicity are important because gametes are only viable for a few hours post-release and sperm dilution rapidly degrades fertilization potential in ocean conditions (Oliver and Babcock, 1992; Levitan and Petersen, 1995; Miller et al., 2016). The only observed timing anomaly was that the 2021 reproduction event occurred a month earlier than expected, which likely represents a case of split spawning. Split spawning in coral populations occurs when gamete maturation and mass spawning are split over two consecutive months and has been observed to occur if the full moon in the primary spawning month occurs either very early or very late in the month (Bastidas et al., 2005; Marhaver et al., 2015; Gilmour et al., 2016; Glynn et al., 2017; Foster et al., 2018). It is hypothesized to help realign reproduction events to favorable environmental conditions (van Woesik et al., 2006; Foster et al., 2018; Hock et al., 2019), increase robustness of coral larval supply and inter-reef connectivity (Hock et al., 2019), and possibly be an evolutionary mechanism that allows corals functioning on a 12-month lunar-driven reproductive cycle to reset their reproductive clocks (Foster et al., 2018). Nonetheless, the 2021 cohort herein still spawned within the predicted NAFM and MAS timeframe. This finding highlights the importance of conducting sexual maturity assessments -and early enough- to catch such events. Missing such events means waiting another year, which can have severe impacts on research and restoration progress. Similar assessments of gravid state can be done for bouldering species by extracting small cores (~1 cm diameter) from a central region of the colony using an underwater drill and then using epoxy to either plug the hole or replace the core after inspection (Koch et al., 2021). Cores can be examined in-situ or brought back to the laboratory for decalcification and dissection.
Consistent with previous work (Miller et al., 2016), we found evidence of genet-specific spawning patterns where some genets spawned earlier and others on the later lunar nights within the predicted window across both years (Figure 7). Genet 62 consistently spawned later, genet 3 spawned earlier, and genet 50 spawned during the three peak nights. However, we also found some genets had more plasticity in spawning timing where in 2020, genet 7 spawned later in the window but earlier in the window in 2021. Similarly, genet 31 spawned earlier in the window in 2020 but later in the window in 2021. Interestingly, both 31 and 7 were held together in the same tank as genet 3 (in 2020 and 2021, respectively), which consistently spawned earlier. This could be evidence of corals using pheromones (endogenous sexual cues) to regulate gamete release (Twan et al., 2006; Caballes and Pratchett, 2017; Koch, 2021a). At the population level, night 6 in 2020 and night 7 in 2021, had the greatest number and diversity of corals that spawned, indicating greater fertilization potential (Levitan et al., 2004; Baums et al., 2013; Miller et al., 2016). In 2021, however, there appeared to be tighter synchronization, and all corals (17/17) and genets (9/9), spawned on night 7, whereas only 33/52 corals and 8/10 genets spawned on night 6 in 2020, indicating that even if the corals split and spawn a month earlier, the synchronization within the window after the full moon can be maintained. The mechanism(s) underlying genet-based versus more plastic responses warrants further investigation. If certain genets never overlap during the spawning window, alternative techniques, such as sperm cryopreservation (Hagedorn et al., 2019), would be necessary to cross them. However, understanding what factors may be driving more plastic responses could potentially be harnessed and manipulated for influencing the timing of gamete release.
4.2 Fertilization
Overall, fertilization was high for crosses and years (~95%) (Figure 8). There was one pair of genets however with no fertilization in either direction (i.e., 34 x 47) (Figure 8A). Gametic incompatibilities can occur, including for two-parent acroporid crosses (Fogarty et al., 2012; Baums et al., 2013; Miller et al., 2018; Chan et al., 2019), indicating the presence of prezygotic isolating mechanisms. Spawning in temporal isolation can be a prezygotic barrier but that does not appear to be the case here since both genets spawned on 5-6 NAFM in 2020 (Figure 7). Other prezygotic barriers could include gamete age (Levitan et al., 2004), or morphological differences between gametes (Baums et al., 2013). Fertilization dynamics in some marine invertebrates are based on protein-mediated egg–sperm interactions (Summers et al., 1975; Vacquier and Moy 1977; Glabe 1985; Galindo et al., 2003; Afonin et al., 2004; Baums et al., 2013). We cannot discount morphological disparities between gametes since those traits were not studied herein, but age is likely not a factor as other crosses conducted on the same night under the same conditions and timeframe did not have failed fertilization. Furthermore, the same set of crosses was repeated two nights in a row to test the reproducibility of our results, and fertilization was still zero on the second attempt.
To test the robustness of our crosses, we conducted selfing controls where eggs were washed of sperm and held in isolation, or were combined with sperm from the same parental genet to see if embryos formed (Figure S7). Self-fertilization (‘selfing’) is an extreme case of inbreeding but is widespread, especially among plants and hermaphroditic invertebrates (Barrett, 2002; Jarne and Auld, 2006). It involves the fusion of male and female gametes from a single genetic individual and represents an evolutionary and reproductive mechanism for isolated individuals to create local populations (Jarne and Charlesworth, 1993). However, a negative consequence of this can be reduced genetic variation and, thus, limited potential for adaptation to environmental change. Selfing may be a viable reproductive strategy for some scleractinian species (Kojis and Quinn, 1981; Brazeau et al., 1998; Gleason et al., 2001; Sherman, 2008), but most, including the major Caribbean reef builders, are partially or entirely reproductively self-incompatible (Heyward and Babcock, 1986; Wallace and Willis, 1994). However, low background levels of selfing have been reported for acroporids (Baums et al., 2005), which we also observed herein where the presence of fertilized eggs was documented for three genets in the No Sperm controls and for six genets in the Sperm + Egg controls (Figure S7). Regardless, mean fertilization success was very low (<5%) in all cases. The presence of fertilized eggs in the No Sperm controls could be attributed to sperm contamination if the washing process was not thorough enough, but rare cases of cell division occurring without sperm fertilization has been reported for another acroporid species (Hagedorn et al., 2019). Even though selfing between sperm and egg occurred for more genets, none of the embryos or larvae proved to be viable. Taken together, these results lead us to be confident in the pedigree of our crosses.
Overall, our spawning and fertilization results provide hope for our restored populations comprised of these same genets where high spawning synchrony and fertilization rates may translate to greater chances of future reproductive success. This idea needs to be corroborated however with in-situ observations of Mote’s restored populations during spawning, which is a goal for extensions of this work. Furthermore, it would be beneficial to test fertilization under prevailing environmental conditions using natural seawater from reefs targeted for restoration. Water quality can impact fertilization (Gilmour, 1999; Omori et al., 2001), and likely differs over spatial and temporal scales, as well as compared to the ultra-filtered sweater used herein. Regardless, our results demonstrate that corals within Mote’s restoration gene pool are highly compatible. Finally, although evaluating broodstock compatibility (via 2-parent crosses) was a specific aim herein, another recommended strategy for maximizing diversity is to conduct batch fertilization among several genets (e.g., 5-10), which may result in more putative sibling groups (i.e., families) (Baums et al., 2019; Koch, 2021b, Koch, 2021c.
4.3 Settlement
4.3.1 Optimizing set-up
Compared to closed containers, we found flow-through settlement bins to be more efficient in terms of maintenance and more effective in terms of settlement (Figures 2-4). Daily water changes in closed tanks require more time and increase the risk of losing larvae, as well as disturbing the CCA dust or larval attachment process. Furthermore, while not tested herein, we surmise that water quality is more stable when larvae are exposed to a continuous influx of fresh seawater during settlement. However, moving forward, we would increase the space between the bins in the raceway, as well as increase the hole and mesh size to ~200um to allow for greater circulation within the settlement bins. Finally, we observed no settlement on the walls or surfaces of the flow-through bins, which can happen in glass tanks. This is not ideal as larvae that settle on surfaces other than the substrates are often not recoverable.
Coral settlement research has shown that larvae also respond to physical cues and may prefer textured substrates with small niches and grooves (presumably for protective reasons) (Whalan et al., 2015; Randall et al., 2021). However, for our restoration purposes, we try to maintain as many single genets as possible, which requires separating out and remounting individual recruits. Non-flat surfaces make this virtually impossible. Hence, if high settlement -without the need to separate out recruits later- is the primary goal, we suggest using textured substrates, but if maintaining as many unique genets as possible is the goal, we recommend using flat surfaces. We have also noticed that fouling mitigation, whether by manual removal or grazers, is less effective on textured surfaces.
4.3.2 Settlement by family
Our settlement results revealed a number of different patterns (Figure 9). First, while we could not statistically compare settlement rates for our 2020 cohort, we could compare relative success across the families and identify ones that needed to be repeated to confirm our results. For a number of 2020 crosses that had no or nominal settlers, we repeated them in 2021 and found that three of the five repeated crosses produced viable settlers and recruits, suggesting other confounding factors that impacted settlement in 2020. As previously mentioned, a bacterial outbreak (spp. unknown) occurred in some of our larval rearing containers which impacted motility. Even though we still achieved settlement across the majority of families, we cannot discount the potential impact this bacterium had on larval fitness, settlement, or post-settlement survival. We surmise overstocked cultures could be a possible explanation, which highlights the importance of larval rearing containers with a high water to air surface ratio to promote gas exchange. We therefore recommend using larval cones to rear larvae which have a large surface area, continuous drip line from above, and aeration from below that gently bubbles and keeps larvae on the surface (Pollock et al., 2017). This method allows for upscaling and more easily rearing large quantities of larvae, with less maintenance than larval cultures in closed containers. Other factors that could have led to failed settlement for some crosses in 2020 include polyspermy, genotypic incompatibilities and postzygotic isolating mechanisms. However, our broodstock was largely compatible in general.
We found several cases where a cross was more -or only- successful in one direction, which has been observed elsewhere (Levitan et al., 2004; Baums et al., 2013). Genets 13 and 50 were crossed in both directions in 2019 and 2020, and we achieved the same result where 13s x 50e produced no or almost no settlers, and the settlers that did occur, perished within months. More striking however was the observation that, in general, any cross with 31 as the dam was largely unsuccessful, which was reproducible across crosses and years (Figures 9, 10). Because fertilization occurred for all crosses and unviability was most noticeable during the process of rearing embryos to larvae, potential explanations include maternal (egg) issues, breakdown in zygotic genome activation, or differences in gene complexes that lead to developmental breakdown depending on when it occurred in relation to blastulation and gastrulation. With limited genotypic diversity remaining in natural populations and restored populations being created largely via asexual -but now sexual- restoration methods, understanding potential constraints on future reproductive potential of restored populations comprised of these genets is important. Especially because genet 31 is known to be a top-performing genet in terms of growth (Bartels, personal obs.), as it consistently has to be fragmented more frequently than other genets in the in-situ asexual propagation nursery because it grows so fast. Furthermore, preliminary results from heat stress experiments indicate genet 31 to be more thermally tolerant (Klepac, unpublished data), but disease resistance research has shown it to be white-band disease susceptible (Foster and Gilmour, 2016; Muller et al., 2018). These findings highlight the importance of considering trade-offs and evaluating a wide range of phenotypic traits when selecting genets to propagate asexually and sexually, but also the importance of producing and outplanting unique genets so that genetic diversity can support more effective selection and adaptive evolution. Moving forward, we will further investigate the viability of genet 31’s eggs by (1) assessing gamete quality (e.g., protein and lipid content), (2) finely tracking embryo and larval development to determine when exactly the breakdown occurs, and (3) conducting more crosses using different genets to see how widespread the issue is.
4.3.3 Settlement by color
Consistent with our prediction, larvae preferentially settled on pink and purple substrates, which we hypothesize is a response to the spectral cues that mimic CCA (Mason et al., 2011; Foster and Gilmour, 2016). CCA is a well-known chemical inducer of larval settlement/metamorphosis (Ritson-Williams et al., 2010; Ritson-Williams et al., 2016; Gomez-Lemos et al., 2018; Jorissen et al., 2021), but it appears larvae are simultaneously integrating different cue types to choose the most appropriate place to settle. This is supported by our finding that larvae settled more on pink and purple substrates when the matching chemical cue of CCA was present but chose white more often when CCA was not present (Figure 11). The use of multiple cue types likely helps to prevent settlement on other objects/organisms that are similar in color to CCA but not actually CCA, such as soft corals (e.g., purple sea fan, Gorgonia ventalina). Furthermore, settlement and sensory biology studies indicate the presence of opsins and olfactory receptors in coral larvae (Mason et al., 2011; Spies et al., 2016; Mason et al., 2021), further supporting the idea that larvae use multiple cue types during settlement. Ultimately, mimicking or replicating the various cues coral larvae use during settlement can help to increase settlement rates and upscale the number of sexually produced corals for restoration.
In the absence of a matching chemical cue, larvae chose white substrates, possibly as they perceived them to be ‘bare’ reef. One study found that coral larvae altered their settlement preferences depending on the age of the benthic community and availability of bare space (Elmer et al., 2018). Bare space may be perceived as the ‘next best’ option where interspecific competition -especially with macro and turf algae- is reduced, which has been shown to negatively impact coral settlement and post-settlement survival (Vermeij et al., 2009; Leong et al., 2018).
Another widely used technique in ASR, that would provide both the chemical and spectral cues associated with CCA -and preclude the need for colored substrates- is conditioning substrates for 1-3 months before settlement so that they are partially or wholly colonized by CCA (Figure S9). This method has been shown to increase settlement and post-settlement survival during ASR of Orbicella faveolata (Koch, unpublished data) and other species (Erwin et al., 2008; Randall et al., 2020). However, there can be trade-offs with this strategy. For example, the CCA that naturally occurs at our facility is quite competitive and can easily overgrow recruits, or slow their growth, as a result of interspecific competition. We noticed that as acroporid recruits grow, they often produce a ‘halo’ of bare space around them, which could indicate a chemical defense. There is evidence that reef corals have the ability to employ offensive or defensive chemicals (e.g., physiochemical barriers) (Sutherland et al., 2004; Ben-Ari et al., 2018). The production of a defensive compound is energetically costly and could therefore take away energy for growth. Further research is needed to test these ideas. Nonetheless, to promote the fastest growth possible, we use CCA for its cues during settlement, and then gently brush it off after metamorphosis to prevent competitive overgrowth.
4.4 Optimizing post-settlement grow-out
We optimized coral grow-out by comparing zooxanthellae acquisition, growth, and survival for recruits reared with -or without- adult corals and additional lighting. With algal symbionts providing most of the coral’s nutritional requirements (Muscatine et al., 1981; Falkowski et al., 1984), the sooner symbiosis is established, the better chances the coral has for survival (Suzuki et al., 2013). While there are different strategies to facilitate the uptake of these critical organisms [e.g., the use of symbiont cultures (Pollock et al., 2017)], we tested whether this process could be accelerated by adding adult corals to our tanks, which naturally shed symbionts in their surface mucus layer (Brown and Bythell, 2005). However, the naturally low light conditions in our indoor laboratory (~14 µmol photons m-2 s-1) are not conducive to maintaining adult corals over the long-term, which can receive in-situ PAR levels of 200-500 (Edmunds et al., 2018). Furthermore, even though too much light too soon can be stressful for early recruits (McMahon, 2018; Kreh, 2019), once symbiosis is established, the coral’s energetic demands increase. Therefore, our light regime was based on previous studies that developed an irradiance regime that slowly increased over time (McMahon, 2018; Kreh, 2019).
Consistent with our predictions, we found recruits reared in the presence of adult corals and under an increasing irradiance regime, had faster zooxanthellae uptake over time and increased growth compared to recruits reared without those two factors (Figure 12). However, there was no difference in median survival time between treatments. The conditions adult corals require for survival and growth are not necessarily going to be the same that early settlers/young recruits need, so further research is necessary to continue to optimize the grow-out process for accelerating restoration-based interventions. Nonetheless, our results highlight the importance of an appropriate lighting scheme and the benefit of facilitating early zooxanthellae uptake. Importantly, budgets may differ across laboratories and while top-of-the-line lights may have more settings and customizable programs, more affordable options exist, which can still provide basic lighting needs that will benefit corals. Ultimately though, faster growth translates to reduced grow-out time, which can save time, money, supplies and effort.
4.5 Active restoration
To promote survival, adaptive potential, and future reproductive success of restored populations, we outplant in a way that takes into consideration density, diversity/genetics (Baums, 2008; Baums et al., 2019; Koch, 2021c), sexual maturation, and recommendations from NOAA’s “Recovery Plan for Elkhorn Coral (Acropora palmata) and Staghorn Coral (A. cervicornis)” (NMFS, 2015). For example, to promote future fertilization success, we outplant dense populations using NOAA’s guidelines (NMFS, 2015). To promote genetic restoration, we outplant a diversity of corals with each outplant representing a different genet, family, or cohort (Figure 6A). On average, a typical restoration-based outplant event comprised of sexual recruits has ~100 novel genets (Table 2). To reduce the time to the onset of sexual reproduction, we outplant multiple clonal fragments of each genet close to one another in a cluster, so that over time, they may fuse to form a larger colony faster and reproduce sooner (Figure 6B) (Koch et al., 2021). This strategy requires more time, space, and resources to fragment and grow the replicate corals, so a parallel strategy we implement is to outplant individual plugs as single genets across different sites. The trade-off with this strategy however appears to be that there may be a higher likelihood of reduced genet diversity over time as the loss of a single plug equates to the loss of that genet (Table 2). Furthermore, we expect sexual maturation of single outplants is slower than clusters, but we still need to confirm this. Thus, we will monitor these outplants over the long-term to effectively compare coral/genet/family survival, as well as timing of sexual maturation, for the different strategies. Nonetheless, which strategy used will also likely depend on specific project goals and availability of personnel, space, time and resources. We estimated the average monthly cost of maintaining ~1,000 corals in a single raceway to be ~$200. This includes items like grazers, food, cleaning supplies, circulation pumps, substrates, and supplies for pest mitigation, inventory management and water quality monitoring. This estimate does not include costs associated with raceway purchase, fragmentation equipment, utilities, seawater treatment, or staff salary.
Data availability statement
The datasets presented in this study can be found in online repositories. The names of the repository/repositories and accession number(s) can be found below: The datasets generated herein were deposited into Dryad. Koch, Hanna R. et al. (2022), Assisted sexual reproduction of Acropora cervicornis for active restoration on Florida’s Coral Reef, Dryad, Dataset, https://doi.org/10.5061/dryad.8kprr4xr2.
Author contributions
HK conceived and designed the study. HK, BM, CL, and CE collected data and reared recruits. HK and BM analyzed results. HK, CL, CE, and EB conducted restoration. EB led fieldwork operations and restoration activities. HK wrote the manuscript. BM, CL, and EB, reviewed the manuscript. All authors contributed to the article and approved the submitted version.
Funding
This work was supported by the NOAA Restoration Center (#NA19NMF4630259), Florida Fish & Wildlife Conservation Commission and Fish & Wildlife Research Institute (#21069), and a Mote Postdoctoral Research Fellowship award to HK.
Acknowledgments
This work was authorized under the following Florida Keys National Marine Sanctuary permits FKNMS-2018-088-A2 and FKNMS-2015-163-A3, as well as Florida Fish & Wildlife Conservation Commission Special Activity Licenses 2019-1724-SCRP and SAL-2020-1724-SCRP. We are grateful to Michael Crosby and Erinn Muller for funding support. We kindly thank Amelia Moura (Coral Restoration Foundation) for assistance in designing the coral spawning nurseries and Keri O’Neil (Florida Aquarium) for helpful discussions. We would like to thank the following people for assisting with coral and/or gamete collections in 2020 and 2021: Nils Ehmke, Kiera Albano, Ellyse Anderson, Yuen Azu, Gregory Bales, Sydney Bell, Summer Brooks, Haley Burleson, Jack Bush, Morgan Chakraborty, Zachary Craig, Gabrielle D’Alonzo, Allison Delashmit, Charles Delashmit, Drew Delashmit, Mason Delashmit, Harlie Falzone, Jessyca Garlock, Madeleine Khoury, Megan Laufer, Kenaro Malcolm, Alicia Manfroy, Kerri Mannino-Cuva, Dakotah Merck, Elizabeth Moore, Merry Moore, Amy Neubauer, Caroline Neubauer, Elizabeth Neubauer, Porter Neubauer, Sibelle O’Donnell, Andria Piekarz, Brooke Pohlman, Amanda Quasunella, Stephanie Sirotzke, Hailey Vaughan, Marina Villoch, and Lydia Wasmer. We would like to thank the following people for fieldwork assistance: Ian Combs, Sarah Hamlyn, Kyle Knoblock, Joe Kuehl, Samantha Simpson, Cory Walter, and the staff of Captain Hooks (Kim Davies Roney, Jeffery Wright, and Jack Gruba). We thank the following for assistance in rearing sexual recruits: Jake Clement, Shayne Doone, Evan Ernst, Gwynne Hayes, Mackenzie Magyar, Mara Riese, and Colleen Shortal.
Conflict of interest
The authors declare that the research was conducted in the absence of any commercial or financial relationships that could be construed as a potential conflict of interest.
Publisher’s note
All claims expressed in this article are solely those of the authors and do not necessarily represent those of their affiliated organizations, or those of the publisher, the editors and the reviewers. Any product that may be evaluated in this article, or claim that may be made by its manufacturer, is not guaranteed or endorsed by the publisher.
Supplementary material
The Supplementary Material for this article can be found online at: https://www.frontiersin.org/articles/10.3389/fmars.2022.959520/full#supplementary-material
References
Acropora Biological Review Team (2005). “Atlantic Acropora status review,” in Report to national marine fisheries service.
Afonin S., Dürr U. H. N., Glaser R. W., Ulrich A. S. (2004). ‘Boomerang’-like insertion of a fusogenic peptide in a lipid membrane revealed by solid-state 19F NMR. Magn. Reson. Chem. 42, 195–203.
Ali A., Kriefall N. G., Emery L. E., Kenkel C. D., Matz M. V., Davies S. W. (2019). Recruit symbiosis establishment and symbiodiniaceae composition influenced by adult corals and reef sediment. Coral Reefs 38, 405–415. doi: 10.1007/s00338-019-01790-z
Amar K. O., Rinkevich B. (2007). A floating mid-water coral nursery as larval dispersion hub: Testing an idea. Mar. Biol. 151, 713–718. doi: 10.1007/s00227-006-0512-0
Ayalon I., Rosenberg Y., Benichou J. I. C., Campos C. L. D., Sayco S. L. G., Nada M. A. L., et al. (2020). Coral gametogenesis collapse under artificial light pollution. Curr. Biol.
Babcock R. C. (1991). Comparative demography of 3 species of scleractinian corals using age-dependent and size-dependent classifications. Ecol. Monogr. 61, 225–244. doi: 10.2307/2937107
Baird A. H., Guest J. R., Willis B. L. (2009). Systematic and biogeographical patterns in the reproductive biology of scleractinian corals. Annu. Rev. Ecol. Evol. S 40, 551–571. doi: 10.1146/annurev.ecolsys.110308.120220
Barrett S. C. H. (2002). The evolution of plant sexual diversity. Nat. Rev. Genet. 3, 274–284. doi: 10.1038/nrg776
Bastidas C., Croquer A., Zubillaga A. L., Ramos R., Kortnik V., Weinberger C., et al. (2005). Coral mass- and split-spawning at a coastal and an offshore Venezuelan reefs, southern Caribbean. Hydrobiologia 541, 101–106. doi: 10.1007/s10750-004-4672-y
Baums I. B. (2008). A restoration genetics guide for coral reef conservation. Mol. Ecol. 17, 2796–2811. doi: 10.1111/j.1365-294X.2008.03787.x
Baums I. B., Baker A. C., Davies S. W., Grottoli A. G., Kenkel C. D., Kitchen S. A., et al. (2019). Considerations for maximizing the adaptive potential of restored coral populations in the western Atlantic. Ecol. Appl. 29. doi: 10.1002/eap.1978
Baums I. B., Devlin-Durante M. K., Polato N. R., Xu D., Giri S., Altman N. S., et al. (2013). Genotypic variation influences reproductive success and thermal stress tolerance in the reef building coral, Acropora palmata. Coral Reefs 32, 703–717. doi: 10.1007/s00338-013-1012-6
Baums I. B., Miller M. W., Hellberg M. E. (2005). Regionally isolated populations of an imperiled Caribbean coral, Acropora palmata. Mol. Ecol. 14, 1377–1390. doi: 10.1111/j.1365-294X.2005.02489.x
Beiring E. A., Lasker H. R. (2000). Egg production by colonies of a gorgonian coral. Mar. Ecol. Prog. Ser. 196, 169–177. doi: 10.3354/meps196169
Ben-Ari H., Paz M., Sher D. (2018). The chemical armament of reef-building corals: Inter- and intra-specific variation and the identification of an unusual actinoporin in Stylophora pistilata. Sci. Rep. 8, 251. doi: 10.1038/s41598-017-18355-1
Boström-Einarsson L., Babcock R. C., Bayraktarov E., Ceccarelli D., Cook N., Ferse S. C. A., et al. (2020). Coral restoration – a systematic review of current methods, successes, failures and future directions. PLoS One 15, e0226631. doi: 10.1371/journal.pone.0226631
Brazeau D. A., Gleason D. F., Morgan M. E. (1998). Self-fertilization in brooding hermaphroditic Caribbean corals: Evidence from molecular markers. J. Exp. Mar. Biol. Ecol. 231, 225–238. doi: 10.1016/S0022-0981(98)00097-5
Brown B. E., Bythell J. (2005). Perspectives on mucus secretion in reef corals. Mar. Ecol. Prog. Ser. 296, 291–309. doi: 10.3354/meps296291
Caballes C. F., Pratchett M. S. (2017). Environmental and biological cues for spawning in the crown-of-thorns starfish. PLoS One 12. doi: 10.1371/journal.pone.0173964
Calle-Trivino J., Cortes-Useche C., Sellares-Blasco R. I., Arias-Gonzalez J. E. (2018). Assisted fertilization of threatened staghorn coral to complement the restoration of nurseries in southeastern Dominican republic. Reg. Stud. Mar. Sci. 18, 129–134. doi: 10.1016/j.rsma.2018.02.002
Chan W. Y., Peplow L. M., van Oppen M. J. H. (2019). Interspecific gamete compatibility and hybrid larval fitness in reef-building corals: Implications for coral reef restoration. Sci. Rep-Uk 9.
Coffroth M. A., Lewis C. F., Santos S. R., Weaver J. L. (2006). Environmental populations of symbiotic dinoflagellates in the genus symbiodinium can initiate symbioses with reef cnidarians. Curr. Biol. 16, R985–R987. doi: 10.1016/j.cub.2006.10.049
Colella M. A., Ruzicka R. R., Kidney J. A., Morrison J. M., Brinkhuis V. B. (2012). Cold-water event of January 2010 results in catastrophic benthic mortality on patch reefs in the Florida keys. Coral Reefs 31, 621–632. doi: 10.1007/s00338-012-0880-5
Craggs J., Guest J. R., Davis M., Simmons J., Dashti E., Sweet M. (2017). Inducing broadcast coral spawning ex situ: Closed system mesocosm design and husbandry protocol. Ecol. Evol. 7, 11066–11078. doi: 10.1002/ece3.3538
Donovan C., Towle E. K., Kelsey H., Allen M., Barkley H. C., Besemer N., et al. (2020). Coral reef condition: A status report for U.S. coral reefs (U.S: CRCP & Science, UoMCfE).
Drury C., Dale K. E., Panlilio J. M., Miller S. V., Lirman D., Larson E. A., et al. (2016). Genomic variation among populations of threatened coral: Acropora cervicornis. BMC Genomics 17. doi: 10.1186/s12864-016-2583-8
Eakin C. M., Morgan J. A., Heron S. F., Smith T. B., Liu G., Alvarez-Filip L., et al. (2010). Caribbean Corals in crisis: Record thermal stress, bleaching, and mortality in 2005. PLoS One 5. doi: 10.1371/journal.pone.0013969
Edmunds P., Tsounis G., Boulon R., Bramanti L. (2018). Long-term variation in light intensity on a coral reef. Coral Reefs 37. doi: 10.1007/s00338-018-1721-y
Elahi R., Edmunds P. J. (2007). Determinate growth and the scaling of photosynthetic energy intake in the solitary coral Fungia concinna (Verrill). J. Exp. Mar. Biol. Ecol. 349, 183–193. doi: 10.1016/j.jembe.2007.05.007
Elmer F., Bell J. J., Gardner J. P. A. (2018). Coral larvae change their settlement preference for crustose coralline algae dependent on availability of bare space. Coral Reefs 37, 397–407. doi: 10.1007/s00338-018-1665-2
Enochs I. C., Manzello D. P., Carlton R., Schopmeyer S., van Hooidonk R., Lirman D. (2014). Effects of light and elevated pCO(2) on the growth and photochemical efficiency of Acropora cervicornis. Coral Reefs 33, 477–485.
Erwin P. M., Song B., Szmant A. M. (2008). “Settlement behavior of Acropora palmata planulae: Effects of biofilm age and crustose coralline algal cover,” in Proceedings of the 11th International Coral Reef Symposium, Lauderdale, Florida.
Falkowski P. G., Dubinsky Z., Muscatine L., Porter J. W. (1984). Light and the bioenergetics of a symbiotic coral. Bioscience 34, 705–709. doi: 10.2307/1309663
Fogarty N. D., Vollmer S. V., Levitan D. R. (2012). Weak prezygotic isolating mechanisms in threatened Caribbean acropora corals. PLoS One 7. doi: 10.1371/journal.pone.0030486
Foster T., Gilmour J. P. (2016). Seeing red: Coral larvae are attracted to healthy-looking reefs. Mar. Ecol. Prog. Ser. 559, 65–71. doi: 10.3354/meps11902
Foster T., Gilmour J. (2020). Egg size and fecundity of biannually spawning corals at Scott reef. Sci. Rep-Uk 10.
Foster T., Heyward A. J., Gilmour J. P. (2018). Split spawning realigns coral reproduction with optimal environmental windows. Nat. Commun. 9. doi: 10.1038/s41467-018-03175-2
Galindo B. E., Vacquier V. D., Swanson W. J. (2003). Positive selection in the egg receptor for abalone sperm lysin. Proc. Natl. Acad. Sci. U.S.A. 100, 4639–4643.
Gardner T. A., Cote I. M., Gill J. A., Grant A., Watkinson A. R. (2003). Long-term region-wide declines in Caribbean corals. Science 301, 958–960. doi: 10.1126/science.1086050
Gardner T. A., Cote I. M., Gill J. A., Grant A., Watkinson A. R. (2005). Hurricanes and Caribbean coral reefs: Impacts, recovery patterns, and role in long-term decline. Ecology 86, 174–184. doi: 10.1890/04-0141
Gignoux-Wolfsohn S. A., Marks C. J., Vollmer S. V. (2012). White band disease transmission in the threatened coral, Acropora cervicornis. Sci. Rep-Uk 2.
Gignoux-Wolfsohn S. A., Precht W. F., Peters E. C., Gintert B. E., Kaufman L. S. (2019). Ecology, histopathology, and microbial ecology of a white-band disease outbreak in the threatened staghorn coral Acropora cervicornis. Dis. Aquat Organ 137, 217–237. doi: 10.3354/dao03441
Gilmour J. (1999). Experimental investigation into the effects of suspended sediment on fertilisation, larval survival and settlement in a scleractinian coral. Mar. Biol. 135, 451–462. doi: 10.1007/s002270050645
Gilmour J., Speed C. W., Babcock R. (2016). Coral reproduction in Western Australia. Peerj 4. doi: 10.7717/peerj.2010
Glabe C. G. (1985). Interaction of the sperm adhesive protein, bindin, with phospholipid vesicles. i. specific association of bindin with gel-phase phospholipid vesicles. J. Cell Biol. 100, 794–799.
Gleason D. F., Brazeau D. A., Munfus D. (2001). Can self-fertilizing coral species be used to enhance restoration of Caribbean reefs? B Mar. Sci. 69, 933–943.
Glynn P. W., Colley S. B., Carpizo-Ituarte E., Richmond R. H. (2017). “Coral reproduction in the Eastern pacific,” in Coral reefs of the Eastern tropical pacific: Persistence and loss in a dynamic environment, vol. 8. , 435–476.
Goergen E. A., Moulding A. L., Walker B. K., Gilliam D. S. (2019). Identifying causes of temporal changes in Acropora cervicornis populations and the potential for recovery. Front. Mar. Sci. 6. doi: 10.3389/fmars.2019.00036
Gomez-Lemos L. A., Doropoulos C., Bayraktarov E., Diaz-Pulido G. (2018). Coralline algal metabolites induce settlement and mediate the inductive effect of epiphytic microbes on coral larvae. Sci. Rep-Uk 8.
Graus R. R., Macintyre I. G. (1998). Global warming and the future of Caribbean coral reefs. Carbonate Evaporite 13, 43–47. doi: 10.1007/BF03175433
Hagedorn M., Page C., O'Neil K., Flores D., Tichy L., Chamberland V. F., et al. (2019). Successful demonstration of assisted gene flow in the threatened coral Acropora palmata across genetically-isolated Caribbean populations using cryopreserved sperm. bioRxiv.
Hall V. R., Hughes T. P. (1996). Reproductive strategies of modular organisms: Comparative studies of reef-building corals. Ecology 77, 950–963. doi: 10.2307/2265514
Harrison P. L. (2011). “Sexual reproduction of scleractinian corals,” in Coral reefs: An ecosystem in transition, 59–85.
Hemond E. M., Vollmer S. V. (2010). Genetic diversity and connectivity in the threatened staghorn coral (Acropora cervicornis) in Florida. PLoS One 5. doi: 10.1371/journal.pone.0008652
Heyward A. J., Babcock R. C. (1986). Self-fertilization and cross-fertilization in scleractinian corals. Mar. Biol. 90, 191–195. doi: 10.1007/BF00569127
Hock K., Doropoulos C., Gorton R., Condie S. A., Mumby P. J. (2019). Split spawning increases robustness of coral larval supply and inter-reef connectivity. Nat. Commun. 10.
Hughes T. P. (1994). Catastrophes, phase-shifts, and large-scale degradation of a Caribbean coral-reef. Science 265, 1547–1551. doi: 10.1126/science.265.5178.1547
Hughes T. P., Tanner J. E. (2000). Recruitment failure, life histories, and long-term decline of Caribbean corals. Ecology 81, 2250–2263. doi: 10.1890/0012-9658(2000)081[2250:RFLHAL]2.0.CO;2
Huntington B. E., Miller M. W. (2014). Location-specific metrics for rapidly estimating the abundance and condition of the threatened coral Acropora cervicornis. Restor. Ecol. 22, 299–303. doi: 10.1111/rec.12057
Jarne P., Auld J. R. (2006). Animals mix it up too: The distribution of self-fertilization among hermaphroditic animals. Evolution 60, 1816–1824. doi: 10.1111/j.0014-3820.2006.tb00525.x
Jarne P., Charlesworth D. (1993). The evolution of the selfing rate in functionally hermaphrodite plants and animals. Annu. Rev. Ecol. Syst. 24, 441–466. doi: 10.1146/annurev.es.24.110193.002301
Jorissen H., Galand P. E., Bonnard I., et al. (2021). Coral larval settlement preferences linked to crustose coralline algae with distinct chemical and microbial signatures. Sci. Rep-Uk 11, 14610.
Kemp D. W., Colella M. A., Bartlett L. A., Ruzicka R. R., Porter J. W., Fitt W. K. (2016). Life after cold death: Reef coral and coral reef responses to the 2010 cold water anomaly in the Florida keys. Ecosphere 7. doi: 10.1002/ecs2.1373
Kiel C., Huntington B., Miller M. (2012). Tractable field metrics for restoration and recovery monitoring of staghorn coral Acropora cervicornis. Endanger Species Res. 19, 171–176. doi: 10.3354/esr00474
Koch H. R. (2021a). Can direct sperm exposure be used as a tool to induce female corals to spawn? B Mar. Sci. 97, 129–130. doi: 10.5343/bms.2020.0057
Koch H. R. (2021b). “Chapter 9: Assisted evolution and coral reef resilience,” in Active coral reef restoration: Techniques for a changing planet. Ed. Vaughan D. (J. Ross Publishing).
Koch H. R. (2021c). “Chapter 10: Genetic considerations for coral reef restoration,” in Active coral reef restoration: Techniques for a changing planet. Ed. Vaughan D. (J. Ross Publishing).
Koch H. R., Azu Y., Bartels E., Muller E. M. (2022). No apparent cost of disease resistance on reproductive output in Acropora cervicornis genets used for active coral reef restoration in Florida. Front. Mar. Sci. 9, 958500. doi: 10.3389/fmars.2022.958500
Kojis B. L., Quinn N. J. (1981). Aspects of sexual reproduction and larval development in the shallow-water hermatypic coral, Goniastrea-australensis (Edwards and haime 1857). B Mar. Sci. 31, 558–573.
Kreh P. D. (2019). Optimizing lighting regimes for rearing orbicella faveolata and acropora cervicornis recruits (Nova Southeastern University).
Kuffner I. B., Lidz B. H., Hudson J. H., Anderson J. S. (2015). A century of ocean warming on Florida keys coral reefs: Historic In situ observations. Estuar. Coast. 38, 1085–1096. doi: 10.1007/s12237-014-9875-5
Leong R. C., Marzinelli E. M., Low J., Bauman A. G., Lim E. W., Lim C. Y., et al. (2018). Effect of coral-algal interactions on early life history processes in Pocillopora acuta in a highly disturbed coral reef system. Front. Mar. Sci. 5. doi: 10.3389/fmars.2018.00385
Levitan D. R., Boudreau W., Jara J., Knowlton N. (2014). Long-term reduced spawning in Orbicella coral species due to temperature stress. Mar. Ecol. Prog. Ser. 515, 1–10. doi: 10.3354/meps11063
Levitan D. R., Fogarty N. D., Jara J., Lotterhos K. E., Knowlton N. (2011). Genetic, spatial, and temporal components of precise spawning synchrony in reef building corals of the Montastraea annularis species complex. Evolution 65, 1254–1270. doi: 10.1111/j.1558-5646.2011.01235.x
Levitan D. R., Fukami H., Jara J., Kline D., McGovern T. M., McGhee K. E., et al. (2004). Mechanisms of reproductive isolation among sympatric broadcast-spawning corals of the Montastrea annularis complex. Evolution 58, 308–323. doi: 10.1111/j.0014-3820.2004.tb01647.x
Levitan D. R., Petersen C. (1995). Sperm limitation in the sea. Trends Ecol. Evol. 10, 228–231. doi: 10.1016/S0169-5347(00)89071-0
Levy G., Shaish L., Haim A., Rinkevich B. (2010). Reef restoration: Testing the novel concept of "coral gardening". Isr. J. Ecol. Evol. 56, 90–90.
Lirman D., Thyberg T., Herlan J., Hill C., Young-Lahiff C., Schopmeyer S., et al. (2010). Propagation of the threatened staghorn coral Acropora cervicornis: methods to minimize the impacts of fragment collection and maximize production. Coral Reefs 29, 729–735. doi: 10.1007/s00338-010-0621-6
Lohr K. E., Patterson J. T. (2017). Intraspecific variation in phenotype among nursery-reared staghorn coral Acropora cervicornis (Lamarck 1816). J. Exp. Mar. Biol. Ecol. 486, 87–92. doi: 10.1016/j.jembe.2016.10.005
Marhaver K., Chamberland V., Fogarty N. (2017a). Caribbean Coral spawning for research and restoration (Coral Restoration Consortium & Reef Resilience Network).
Marhaver K., Chamberland V., Fogarty N., Network, RR (2017b). Coral spawning research & larval propagation (Coral Restoration Consortium).
Marhaver K. L., Vermeij M. J. A., Medina M. M. (2015). Reproductive natural history and successful juvenile propagation of the threatened Caribbean pillar coral Dendrogyra cylindrus. BMC Ecol. 15. doi: 10.1186/s12898-015-0039-7
Mason B., Beard M., Miller M. W. (2011). Coral larvae settle at a higher frequency on red surfaces. Coral Reefs 30, 667–676. doi: 10.1007/s00338-011-0739-1
Mason B., Schmale M., Gibbs P., Miller M. W., Wang Q., et al. (2021). Evidence for multiple phototransduction pathways in a reef-building coral. PLoS One 7, e50371.
McMahon N. J. (2018). Optimization of light irradiance during early life of sexually-produced porites astreoides and agaricia agaricites recruits (Nova Southeastern University).
Miller M. W., Baums I. B., Pausch R. E., Bright A. J., Cameron C. M., Williams D. E., et al. (2018). Clonal structure and variable fertilization success in Florida keys broadcast-spawning corals. Coral Reefs 37, 239–249. doi: 10.1007/s00338-017-1651-0
Miller M. W., Williams D. E., Fisch J. (2016). Genet-Specific spawning patterns in Acropora palmata. Coral Reefs 35, 1393–1398. doi: 10.1007/s00338-016-1472-6
Muller E. M., Bartels E., Baums I. B. (2018). Bleaching causes loss of disease resistance within the threatened coral species Acropora cervicornis. Elife 7. doi: 10.7554/eLife.35066.028
Muscatine L., Mccloskey L. R., Marian R. E. (1981). Estimating the daily contribution of carbon from zooxanthellae to coral animal respiration. Limnol Oceanogr 26, 601–611. doi: 10.4319/lo.1981.26.4.0601
Nitschke M. R., Davy S. K., Ward S. (2016). Horizontal transmission of symbiodinium cells between adult and juvenile corals is aided by benthic sediment. Coral Reefs 35, 335–344. doi: 10.1007/s00338-015-1349-0
NMFS (2015). Recovery plan for elkhorn (Acropora palmata) and staghorn (A. cervicornis) corals (Silver Spring, MD: National Marine Fisheries Service), 167.
NOAA (2020). Restoring seven iconic reefs: A mission to recover the coral reefs of the Florida keys national oceanic atmospheric association.
Nozawa Y., Isomura N., Fukami H. (2015). Influence of sperm dilution and gamete contact time on the fertilization rate of scleractinian corals. Coral Reefs 34, 1199–1206. doi: 10.1007/s00338-015-1338-3
Nozawa Y., Lin C. H. (2014). Effects of colony size and polyp position on polyp fecundity in the scleractinian coral genus acropora. Coral Reefs 33, 1057–1066. doi: 10.1007/s00338-014-1185-7
Oliver J., Babcock R. (1992). Aspects of the fertilization ecology of broadcast spawning corals - sperm dilution effects and insitu measurements of fertilization. Biol. Bull. 183, 409–417. doi: 10.2307/1542017
Omori M., Fukami H., Kobinata H., Hatta M. (2001). Significant drop of fertilization of acropora corals in 1999. an after-effect of heavy coral bleaching? Limnol Oceanogr 46, 704–706.
Pandolfi J. M., Bradbury R. H., Sala E., Hughes T. P., Bjorndal K. A., Cooke R. G., et al. (2003). Global trajectories of the long-term decline of coral reef ecosystems. Science 301, 955–958. doi: 10.1126/science.1085706
Pollock F. J., Katz S. M., van de Water J. A. J. M., Davies S. W., Hein M., Torda G., et al. (2017). Coral larvae for restoration and research: A large-scale method for rearing Acropora millepora larvae, inducing settlement, and establishing symbiosis. Peerj 5. doi: 10.7717/peerj.3732
Precht W. F., Gintert B. E., Robbart M. L., Fura R., van Woesik R. (2016). Unprecedented disease-related coral mortality in southeastern Florida. Sci. Rep-Uk 6.
Quigley K. M., Bay L. K., Willis B. L. (2018). Leveraging new knowledge of symbiodinium community regulation in corals for conservation and reef restoration. Mar. Ecol. Prog. Ser. 600, 245–253. doi: 10.3354/meps12652
Randall C. J., Giuliano C., Heyward A. J., Negri A. P. (2021). Enhancing coral survival on deployment devices with microrefugia. Front. Mar. Sci. 8. doi: 10.3389/fmars.2021.662263
Randall C. J., Negri A. P., Quigley K. M., Foster T., Ricardo G. F., Webster N. S., et al. (2020). Sexual production of corals for reef restoration in the anthropocene. Mar. Ecol. Prog. Ser. 635, 203–232. doi: 10.3354/meps13206
Ritson-Williams R., Arnold S. N., Paul V. J. (2016). Patterns of larval settlement preferences and post-settlement survival for seven Caribbean corals. Mar. Ecol. Prog. Ser. 548, 127–138. doi: 10.3354/meps11688
Ritson-Williams R., Paul V. J., Arnold S. N., Steneck R. S. (2010). Larval settlement preferences and post-settlement survival of the threatened Caribbean corals Acropora palmata and A. cervicornis. Coral Reefs 29, 71–81. doi: 10.1007/s00338-009-0555-z
Sakai K. (1998). Effect of colony size, polyp size, and budding mode on egg production in a colonial coral. Biol. Bull. 195, 319–325. doi: 10.2307/1543143
Schopmeyer S. A., Lirman D., Bartels E., Gilliam D. S., Goergen E. A., Griffin S. P., et al. (2017). Regional restoration benchmarks for Acropora cervicornis. Coral Reefs 36, 1047–1057. doi: 10.1007/s00338-017-1596-3
Sherman C. D. H. (2008). Mating system variation in the hermaphroditic brooding coral, Seriatopora hystrix. Heredity 100, 296–303. doi: 10.1038/sj.hdy.6801076
Shlesinger T., Loya Y. (2019). Breakdown in spawning synchrony: A silent threat to coral persistence. Science 365, 1002–1004. doi: 10.1126/science.aax0110
Soong K. Y., Lang J. C. (1992). Reproductive integration in reef corals. Biol. Bull. 183, 418–431. doi: 10.2307/1542018
Speare K. E., Adam T. C., Winslow E. M., Lenihan H. S., Burkepile D. E. (2022). Size-dependent mortality of corals during marine heatwave erodes recovery capacity of a coral reef. Global Change Biol. 28, 1342–1358. doi: 10.1111/gcb.16000
Spies N., Richmond R. H., Seneca F., Murphy J., Martinez J., Lyman A. (2016). “Reef scent: How brooded coral larvae from a tough coral smell their way to a new home,” in American Geophysical union: Ocean sciences meeting 2016.
Summers R. G., Hylander B. L., Colwin L. H., Colwin A. L. (1975). The functional anatomy of the echinoderm spermatozoon and its interaction with the egg at fertilization. Am. Zool 15, 523–551.
Sutherland K. P., Porter J. W., Torres C. (2004). Disease and immunity in Caribbean and indo-pacific zooxanthellate corals. Mar. Ecol. Prog. Ser. 266, 273–302. doi: 10.3354/meps266273
Suzuki G., Yamashita H., Kai S., Hayashibara T., Suzuki K., Iehisa Y., et al. (2013). Early uptake of specific symbionts enhances the post-settlement survival of acropora corals. Mar. Ecol. Prog. Ser. 494, 149–158. doi: 10.3354/meps10548
Szmant A. M. (1986). Reproductive ecology of caribbean reef corals. Coral Reefs 5, 43–53. doi: 10.1007/BF00302170
Tebben J., Motti C. A., Siboni N., Tapiolas D. M., Negri A. P., Schupp P. J., et al. (2015). Chemical mediation of coral larval settlement by crustose coralline algae. Sci. Rep-Uk 5.
Twan W. H., Hwang J. S., Lee Y. H., Wu H. F., Tung Y. H., Chang C. F. (2006). Hormones and reproduction in scleractinian corals. Comp. Biochem. Phys. A 144, 247–253. doi: 10.1016/j.cbpa.2006.01.011
Vacquier V. D., Moy G. W. (1977). Isolation of bindin: the protein responsible for adhesion of sperm to sea urchin eggs. Proc. Natl. Acad. Sci. U.S.A. 74, 2456–2460.
van Oppen M. J. H., Oliver J. K., Putnam H. M., Gates R. D. (2015). Building coral reef resilience through assisted evolution. P Natl. Acad. Sci. U.S.A. 112, 2307–2313. doi: 10.1073/pnas.1422301112
van Woesik R., Lacharmoise F., Köksal S. (2006). Annual cycles of solar insolation predict spawning times of Caribbean corals. Ecol. Lett. 9, 390–398. doi: 10.1111/j.1461-0248.2006.00886.x
van Woesik R., Scott W., Aronson R. (2014). Lost opportunities: Coral recruitment does not translate to reef recovery in the Florida keys. Mar. pollut. Bull. 88, 110–117. doi: 10.1016/j.marpolbul.2014.09.017
Vargas-Angel B., Colley S. B., Hoke S. M., Thomas J. D. (2006). The reproductive seasonality and gametogenic cycle of Acropora cervicornis off broward county, Florida, USA. Coral Reefs 25, 110–122. doi: 10.1007/s00338-005-0070-9
Vermeij M. J. A., Sandin S. A. (2008). Density-dependent settlement and mortality structure the earliest life phases of a coral population. Ecology 89, 1994–2004. doi: 10.1890/07-1296.1
Vermeij M. J., Smith J. E., Smith C. M., Vega Thurber R., Sandin S. A. (2009). Survival and settlement success of coral planulae: Independent and synergistic effects of macroalgae and microbes. Oecologia 159, 325–336. doi: 10.1007/s00442-008-1223-7
Wallace C. C. (1985). Reproduction, recruitment and fragmentation in 9 sympatric species of the coral genus acropora. Mar. Biol. 88, 217–233. doi: 10.1007/BF00392585
Wallace C. C., Willis B. L. (1994). Systematics of the coral genus acropora - implications of new biological findings for species concepts. Annu. Rev. Ecol. Syst. 25, 237–262. doi: 10.1146/annurev.es.25.110194.001321
Walton C. J., Hayes N. K., Gilliam D. S. (2018). Impacts of a regional, multi-year, multi-species coral disease outbreak in southeast Florida. Front. Mar. Sci. 5. doi: 10.3389/fmars.2018.00323
Whalan S., Wahab M. A., Sprungala S., Poole A. J., de Nys R. (2015). Larval settlement: The role of surface topography for sessile coral reef invertebrates. PLoS One 10, e0117675. doi: 10.1371/journal.pone.0117675
Whitman T. N., Negri A. P., Bourne D. G., Randall C. J. (2020). Settlement of larvae from four families of corals in response to a crustose coralline alga and its biochemical morphogens. Sci. Rep-Uk 10.
Keywords: coral restoration, coral conservation, coral sexual propagation, coral settlement, sexual recruits, coral rearing, managed breeding
Citation: Koch HR, Matthews B, Leto C, Engelsma C and Bartels E (2022) Assisted sexual reproduction of Acropora cervicornis for active restoration on Florida’s Coral Reef. Front. Mar. Sci. 9:959520. doi: 10.3389/fmars.2022.959520
Received: 01 June 2022; Accepted: 03 November 2022;
Published: 08 December 2022.
Edited by:
Laura Airoldi, University of Padova Chioggia Hydrobiological Station, ItalyReviewed by:
Karen Lynn Neely, Nova Southeastern University, United StatesRocio Garcia Urueña, University of Magdalena, Colombia
Copyright © 2022 Koch, Matthews, Leto, Engelsma and Bartels. This is an open-access article distributed under the terms of the Creative Commons Attribution License (CC BY). The use, distribution or reproduction in other forums is permitted, provided the original author(s) and the copyright owner(s) are credited and that the original publication in this journal is cited, in accordance with accepted academic practice. No use, distribution or reproduction is permitted which does not comply with these terms.
*Correspondence: Hanna R. Koch, hkoch@mote.org